Introduction
Materials and Methods
Plant material and cultivation environment
Treatment of GA inhibitors
Vegetative growth characteristics
Reproductive growth characteristics
Observation of reproductive growth stages
Investigation of flower bud differentiation rate and panicle type
GC-MS and heatmap analyses
Statistical analysis
Results
Temperature and relative humidity changes
Vegetative growth characteristics
Reproductive growth characteristics
Reproductive growth stages
Rate of flower bud differentiation and panicle type
Metabolites profiling
Discussion
Conclusion
Introduction
Currently, South Korea is undergoing a steady rise in the average annual temperature in due to global warming. According to the Korea Meteorological Administration, the average annual temperature increased from 12.9°C in 2022 to 13.7°C in 2023 (https://data.kma.go.kr). As a result, the Korean climate is gradually changing from temperate to subtropical (Lee et al., 2017). In line with this climate change, interest in and production of various subtropical crops is increasing in South Korea (Jeong et al., 2020). Mango (Mangifera indica L.), referred to as the ‘king of fruits’, is one of the world’s most important tropical and subtropical fruits in the family Anacardiaceae (Viruel et al., 2005; Muthuramalingam et al., 2023). Moreover, mango is one of the major subtropical crops, accounting for the largest share of production among subtropical fruits in South Korea (Jeong et al., 2020). The ‘Irwin’ cultivar, mainly cultivated in the southern regions of South Korea, is native to Florida and is currently cultivated in various parts of the world, including South Korea, Taiwan, and Japan (Wang and Shiesh, 1989; Ueda et al., 2000). It has fiberless flesh which is sweet and flavorful. When the fruit ripens, its skin turns a striking red color, resembling an apple, earning it the name “apple mango.” For these reasons, the number of mango growers is increasing in South Korea with the increase in mango consumption (Jeong et al., 2020). However, now all mango growers always have a successful harvest season.
The optimum growth temperature for mango trees is approximately 20 to 30°C from February to October, when they flower and grow vegetatively (Nunez-Elisea et al., 1996). The temperature required for flower bud differentiation, which determines successful flowering, from the end of October to the end of January, is approximately 5 to 15°C (Ramírez and Davenport, 2010). Because it is impossible for mango trees to grow outside due to the severe winters in South Korea, it is essential to use a greenhouse and a heating system to maintain an appropriate temperature range (Lim et al., 2016). However, even with considerable capital investments in greenhouse cultivation to meet these conditions, the problem of restricted flower bud differentiation remains. Poor flower bud differentiation can lead to problems such as low flowering and fruit set rates. Environmental factors such as the temperature and humidity and amount of light along with tree-related factors such as age, nutrients and hormones, as well as pests and diseases, are all related to poor flower bud differentiation. However, if flower bud differentiation does not occur even when pests and diseases are controlled and environmental factors are met, the problem may reside with the plant itself. According to earlier studies in the literature, excessive gibberellic acid (GA) in mango trees inhibits flower bud differentiation (Kachru et al., 1971; Tomer, 1984). Based on these early findings, several studies have attempted to induce flower bud differentiation via treatments with GA biosynthesis inhibitors such as paclobutrazol (PBZ), prohexadione-calcium (Pro-Ca), and uniconazole (Benavides et al., 2005; Chusri et al., 2008; Naveena Kumara et al., 2023). Moreover, brassinosteroid, a phytohormone, has been proposed as a material to ameliorate the issue of reduced fruit set in mango (Tepkaew et al., 2022).
PBZ, well known around the world and widely used in commercial agriculture, is a growth regulator with various mechanisms. For example, it inhibits GA, increases cytokinin, induces flowering, improves fruit quality, and inhibits vegetative growth (Desta and Amare, 2021; Orozco-Meléndez et al., 2022). PBZ is a triazole-based substance which inhibits endogenous GA biosynthesis by inhibiting the oxidation of ent-kaurene, a GA metabolite, to ent-kaurenoic acid in the GA biosynthesis pathway in plants (Desta and Amare, 2021). However, studies have confirmed the soil persistence and xylem accumulation of PBZ, and Pro-Ca has been indicated as a means of compensating for these problems (Singh and Bhattacherjee, 2005).
Pro-Ca has various mechanisms similar to those of PBZ; it can induce flowering, suppress GA, prevent damage caused by stress, and improve fruit quality. Pro-Ca has a mechanism to reduce endogenous GA by controlling the activity of GA20, an inactive GA, in plants and stopping active GA1 synthesis (Evans et al., 1999). In addition, unlike PBZ, Pro-Ca is decomposed into water and carbon dioxide at a rapid rate by soil microorganisms, mitigating concerns over soil residue and environmental pollution (Rademacher, 2000).
While extensive research has uncovered the pivotal roles of GA in the growth and development of plants, there are relatively few investigations of the specific impacts of GA inhibitors on mango trees, specifically the ‘Irwin’ cultivar. Thus far, investigations have mostly focused on the effects of GA treatments without exploring the subsequent changes in plant substances and metabolites related to flowering. Taking this important topic and the related gap in the literature into consideration, the goal of the present study is to understand the roles of GA inhibitors in vegetative growth and flowering induction in “Irwin” mangoes. A second aim is to obtain uniform bud break and to stabilize flower induction with PBZ and Pro-Ca treatments throughout all stages of bud differentiation, flowering, and the production of ‘Irwin’ mango fruits under greenhouse conditions. To do this, we monitored changes in the temperature and relative humidity in the greenhouse during the treatment conditions and conducted vegetative growth and flowering induction analyses. We also undertook metabolite profiling during the period of flower bud differentiation by gas chromatography-mass spectrometry (GC-MS). This study will provide important information to those who explore the complex molecular mechanisms associated with flower and fruit development in mangoes.
Materials and Methods
The experiment was conducted in a double-layer greenhouse located on the Naedong campus of Gyeongsang National University (35°09’16”N 128°05’11”E) in Jinju, Republic of Korea. The experimental greenhouse was a double-layered plastic greenhouse installed inside a glass greenhouse. The experimental period was from April 29, 2022, to March 31, 2023, encompassing one vegetative growth stage and one reproductive growth stage, and potted mango trees were used.
Plant material and cultivation environment
Two-year-old ‘Irwin’ mango trees were planted in 40-L pots. The selected trees had not flowered or fruited during the previous year. The potting soil consisted of a mixture of masato, perlite, and peat moss at a ratio of 3:1:1 (v:v:v). Following planting, direct watering was conducted for two weeks to soak the soil thoroughly so as to promote proper root establishment. For subsequent irrigation, a nutrient solution containing N, P, and K fertilizers and nitrate was supplied to maintain optimal electrical conductivity (EC) of 0.8 and a pH of 6.0 using an automated nutrient solution dispenser (WIN-7000S, Woosung Hitec Co. Ltd., Yangsan, Republic of Korea). During the vegetative growth stage, the trees were irrigated with 1.5 L of water per day, while during the reproductive growth stage, 1 L of water was applied.
Mango flower differentiation requires a cold winter environment. In Korea, the main flowering induction period is from mid-November to mid-January. The optimum temperature for flowering induction is 10 to 15°C. Temperatures below 5°C can induce growth retardation and cold injury, while temperatures above 20°C can trigger a switch to vegetative growth (Ramírez and Davenport, 2010). Therefore, in this experiment, a heated environment was created using a thermal curtain and an electric fan to maintain the optimum temperature for flowering induction. The temperature and humidity were monitored using a data logger (MX2302A, HOBO, Bourne, MA, USA).
Treatment of GA inhibitors
The plant growth retardants PBZ and Pro-Ca were employed as GA inhibitors. Five trees were randomly selected for each treatment group, resulting in a total of 25 trees. The trees were categorized into the five following groups: control group, PBZ once, PBZ twice, Pro-Ca once, and Pro-Ca twice. PBZ was applied at a concentration of 22.9% as a suspension concentrate formulation at 1,500 ppm, and Pro-Ca was applied at a concentration of 20% as a suspension concentrate formulation at 500 ppm (Chusri et al., 2008; Pérez Barraza et al., 2016). The application was performed on July 21, 2022, at the point when the newly emerged shoots after pruning had reached an average hardening stage. The first treatment was administered on September 27, 2022 with the second treatment applied two weeks later on October 11, 2022.
Vegetative growth characteristics
To investigate the vegetative growth characteristics in mango, leaf and shoot parameters were measured during the early stages of vegetative growth, specifically after the hardening of the shoots in the GA inhibitor treatment groups and at the respective treatment timings. Representative samples of average sized leaves and shoots were selected for these measurements. Leaf length and width were measured using a ruler. Leaf length represented the longest vertical dimension of the leaf, while leaf width was defined as the longest horizontal dimension perpendicular to the leaf length. Shoot thickness and length were measured using a Vernier caliper (M500-181-30, Mitutoyo, Tsukuba, Japan). Stem length was measured from the base to the tip of the internode and thickness was measured 1 cm above the starting point of the internode.
Reproductive growth characteristics
Reproductive growth characteristics in mango were investigated at the full bloom stage, i.e., when floral bud development was completed. The parameters assessed were the panicle length and thickness. Inflorescence length was measured using a ruler, while the corresponding thickness was determined 1 cm above the starting point of the internode using a Vernier caliper.
Observation of reproductive growth stages
Delgado et al. (2011) reported that there were seven reproductive growth stages of mango (Fig. 1). The details of these reproductive stages are given in Table 1. Here, panicle development between the treatments was compared at the dates at which Stages 2, 5, and 7 were reached, as these stages exhibited distinct differences in subsequent growth.
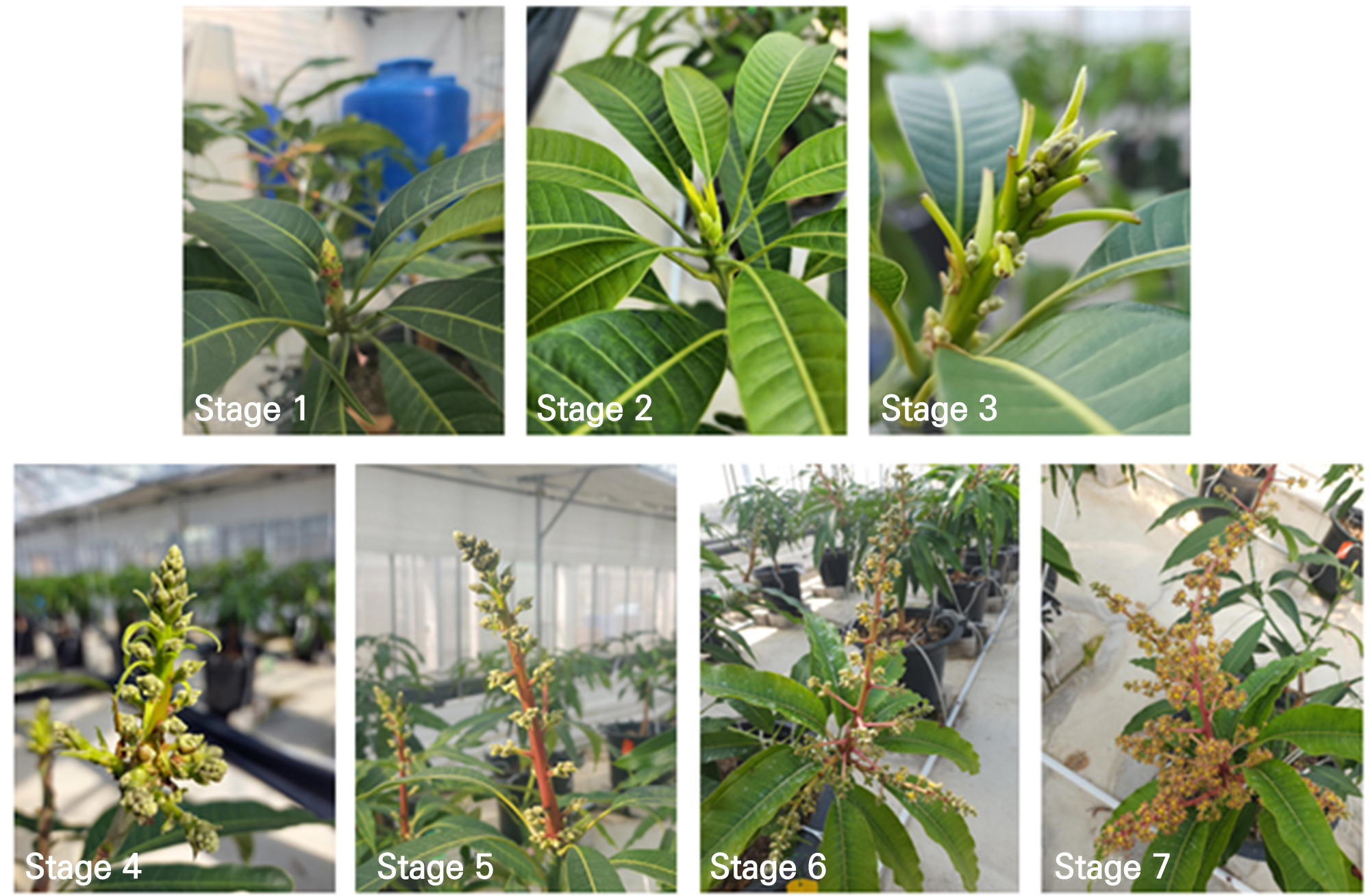
Fig. 1.
Stages of reproductive growth of mango trees: Stage 1 - swelling terminal bud; Stage 2 - emerging bracts; Stage 3 - flower buds are visible and lateral buds occur; Stage 4 - panicles begin to elongate; Stage 5 - much more elongated panicle; Stage 6 - panicles are fully shaped and begin to bloom; Stage 7 - full bloom (over 50% bloomed).
Table 1.
Classification of reproductive growth stages according to each characteristic
Investigation of flower bud differentiation rate and panicle type
The flower bud differentiation rate was expressed as the ratio of the number of panicles generated through the inflorescence primordia in the entire apical part of the last shoots on each tree. The inflorescence types were classified into four stages: flower, leaf, mixed, and non-occurred (Chusri et al., 2008).
GC-MS and heatmap analyses
Leaf samples to be analyzed were obtained from the leaves immediately below the inflorescence during the reproductive growth period, specifically before the emergence of the terminal bud and at Stages 1, 4, and 6. The collected leaf samples were rapidly frozen in liquid nitrogen and stored at ‒80°C. Prior to the GC-MS analysis, each frozen leaf sample was pulverized using a mortar and pestle. Subsequently, 3 g aliquots of the powdered samples were transferred to 30-mL glass tubes. Into each tube, an amount of 10 mL of 80% methyl alcohol (MeOH) (Samchun Pure Chemical Co. Ltd., Pyeongtaek, Republic of Korea) was added, followed by vortex mixing using a Vortex-Genie 2 mixer (Scientific Industries Inc., Bohemia, NY, USA). To prevent exposure to light, the tubes were wrapped in foil and placed on an incubated shaker (IST-4075R, Jeio Tech Co. Ltd., Daejeon, Republic of Korea) for 48 h.
Following the shaking period, the supernatant was filtered through Whatman No. 1 filter paper (1001-110, Whatman, UK). The filtrate was then evaporated on a clean bench (777A2, CHC LAB Co. Ltd., Daejeon, KOREA) until only a dry powder remained. Then, 1 mg of dry powder was diluted with 1 mL of 80% MeOH and transferred to a 2-mL vial (5182-0716, Agilent Technologies, Santa Clara, CA, USA) for the subsequent analysis.
To validate the enhanced levels of metabolites in the mango leaf extracts, a GC-MS analysis was conducted. This analysis was carried out using an Agilent GC8890-MSD5977B (Agilent Technologies, Santa Clara, CA, USA) instrument equipped with a HP-5ms column (Agilent Technologies, Santa Clara, CA, USA) with a length of 30 m, an internal diameter of 0.25 mm, and a film thickness of 0.25 microns. The flow rate was set to 1.7 mL·min-1 in the splitless mode. The oven temperature was initially raised at a rate of 40°C·min-1, followed by a ramp rate of 12°C·min-1 until the temperature reached 280°C, where it was held for 2 min. The total run time for the analysis was 30 min.
The metabolites and their respective peak areas in the various treatments, which represent quantitative values obtained from the metabolites through the GC-MS analysis, were subjected to the MetaboAnalyst 5.0 online tool (http://www.metaboanalyst.ca) to generate a heatmap with default parameters.
Statistical analysis
The vegetative and panicle characteristics, mango panicle stages, rate of flower bud differentiation, and panicle type percentages were statistically analyzed using the statistical software SAS version 9.4 (SAS Institute Inc., Cary, NC, USA). All investigations were conducted with five repetitions. The statistical significance of the results was determined using Duncan’s multiple range test (p < 0.05). All graphs were generated using SigmaPlot 12.3 (Systat Software Inc., San Jose, CA, USA).
Results
Temperature and relative humidity changes
Greenhouse temperatures and humidity levels were monitored from May 1st to October 21st, coinciding with the nutritional growth stage of mangoes and the onset of fruit development (Fig. 2). During this period, the average temperature inside the facility was effectively maintained within the optimal range of approximately 20–30°C. Although occasional spikes in humidity due to summer rainfall were observed, the relative humidity remained within the suitable range of around 60–70% for most of the period. A comparison of internal and external greenhouse temperatures during this period revealed a consistent temperature difference of approximately 10°C, indicating the effectiveness of the greenhouse in maintaining optimal temperature conditions for mango growth (Fig. 3).
Subsequent temperature and humidity monitoring was conducted from November 15, 2022, to January 15, 2023 during the mango’s flowering induction stage (Fig. 4). The average temperature during this period was approximately 13.4°C. The average relative humidity was approximately 62%, within the suitable humidity range for the optimal growth of mangoes.
Vegetative growth characteristics
Assessments of vegetative growth characteristics included the leaf length, leaf width, shoot length, and shoot thickness (Table 2). No significant differences were observed in the leaf length or leaf width between the control and treatment groups. Similarly, no significant differences were found in the shoot length and thickness.
Table 2.
Vegetative growth characteristics of ‘Irwin’ mango on October 21, 2022
Treatment | Leaf length (cm) | Leaf width (cm) | Shoot length (cm) | Shoot width (mm) |
Control | 20.44 ± 0.70 az | 4.66 ± 0.23 a | 13.05 ± 2.63 a | 6.94 ± 0.19 a |
PBZ once | 19.69 ± 1.23 a | 4.40 ± 0.10 a | 14.68 ± 2.32 a | 7.09 ± 0.84 a |
PBZ twice | 20.35 ± 1.16 a | 4.68 ± 0.18 a | 14.45 ± 3.67 a | 7.19 ± 0.63 a |
Pro-Ca once | 20.67 ± 1.67 a | 4.60 ± 0.22 a | 13.93 ± 2.63 a | 7.86 ± 0.87 a |
Pro-Ca twice | 20.13 ± 0.53 a | 4.59 ± 0.31 a | 16.10 ± 3.57 a | 7.81 ± 0.79 a |
Reproductive growth characteristics
Panicle characteristics were measured at full bloom, signifying the completion of inflorescence development. No significant differences were detected in the inflorescence length or thickness between the control and treatment groups (Table 3).
Table 3.
Panicle characteristics of ‘Irwin’ mango on March 17, 2023
Treatment | Panicle length (cm) | Panicle width (mm) |
Control | 15.29 ± 6.5 az | 9.09 ± 1.6 a |
PBZ once | 16.90 ± 6.9 a | 9.97 ± 1.6 a |
PBZ twice | 22.25 ± 5.9 a | 9.72 ± 1.5 a |
Pro-Ca once | 20.39 ± 7.3 a | 8.98 ± 1.4 a |
Pro-Ca twice | 13.62 ± 4.9 a | 9.20 ± 1.4 a |
Reproductive growth stages
Reproductive growth stages were classified according to the number of days required to reach stages 2, 5, and 7 (Table 4). At stage 2, no significant differences were observed between the control group and any of the Pro-Ca treatment groups. However, within the PBZ treatment groups, the group treated once exhibited a significant advancement of eight days, while the group treated twice demonstrated a significant advancement of six days compared to the control group. Regarding stage 5, only the PBZ twice treatment group showed a significant difference, advancing by four days compared to the control group. No significant differences were observed between the remaining treatment groups. Finally, at stage 7, corresponding to full bloom, no significant differences were observed between the control group and all treatment groups (Table 4).
Table 4.
Panicle development of 2, 5, 7 stages in ‘Irwin’ mango
Treatment | Stage 2 | Stage 5 | Stage 7 |
Control | Feb 03 az | Mar 12 a | Mar 22 a |
PBZ once | Jan 26 b | Mar 09 ab | Mar 22 a |
PBZ twice | Jan 28 b | Mar 08 b | Mar 22 a |
Pro-Ca once | Jan 30 ab | Mar 09 ab | Mar 22 a |
Pro-Ca twice | Jan 30 ab | Mar 11 ab | Mar 23 a |
Rate of flower bud differentiation and panicle type
Flower bud differentiation was expressed as a percentage of the number of panicles formed relative to the number of stems capable of producing panicles in each tree (Fig. 5). The control group exhibited a flower bud differentiation rate of 77.5%, and the PBZ once treatment showed a rate of 94.5%, with no significant difference between the two groups. However, the flower bud differentiation rates for the PBZ twice treatment and both Pro-Ca treatments reached 100%, indicating a significant increase compared to the control group.
Subsequently, the panicle form was classified into four types: flower, leaf, mixed, and non-occurred. In this experiment, all panicles observed were of the mixed type, with the flower and leaf types absent. In addition, 22.5% of the control group and 5.5% of the group treated with PBZ once remained dormant or failed to form any buds (Fig. 6).
Metabolites profiling
Metabolite profiling was performed on leaf samples collected from shoots directly below the apical shoot, where panicle formation was expected. Samples were collected at four time points, as follows: (1) before bud emergence, (2) at stage 1 of panicle development, (3) at stage 4, and (4) at stage 6. Each stage corresponded to specific dates: December 19, 2022 (1), January 19, 2023 (2), March 3, 2023 (3), and March 16, 2023 (4). The first, second, third, and fourth sets of samples contained 15, 18, 23, and 25 identified compounds, respectively. Eight compounds were consistently present across all stages: one saturated fatty acid, three phenolic compounds, and one antioxidant compound (Suppl. Table S1). Notably, four of these compounds (furfuryl alcohol, syringol, DDMP, and catechol) exhibited decreasing levels as the flowering stage progressed (Fig. 7). Conversely, palmitic acid, pyrogallol, benzoic acid, and hydroxymethylfurfural all displayed steady increases throughout the reproductive growth period (Fig. 7).
Beyond the eight ubiquitous compounds, three emerged exclusively from stage 1 onwards, while one vanished upon flowering onset. Specifically, the pyrimidine derivative 4,5-diamino-2-hydroxypyrimidin was identified at stage 1 and persisted until flowering commenced, after which it became undetectable. Conversely, the remaining three compounds, dihydroconiferyl alcohol, phytol, and lupeol, debuted at stage 4 (Suppl. Table S2). Absent in earlier stages, these compounds first appeared at the “Panicles begin to elongate” (stage 4). Notably, lupeol and phytol displayed a clear increase in the treatment groups compared to the control group (Fig. 8).
Discussion
Global warming has led to rising average temperatures, including Korea, prompting a surge in tropical crop cultivation. Among these, mangoes have exhibited the most substantial growth (Jeong et al., 2020). However, inconsistent flowering induction poses a significant threat to fruit set and yield, jeopardizing farmers’ economic profit. This study aimed to enhance mango flowering by inhibiting the accumulation of gibberellic acid (GA), a plant hormone impeding vegetative growth, through GA inhibitors.
The experiment was conducted from April 29, 2022, to March 31, 2023, within a double-layer greenhouse (plastic and glass) located on the Naedong campus of Gyeongsang National University in Jinju, South Korea. Temperature and humidity were rigorously monitored to optimize both vegetative and reproductive growth of the mangoes.
During the vegetative growth period (May 1 to October 21, 2022), the greenhouse temperature was consistently maintained between 20 and 30°C, aligning with optimal mango growth conditions (Nunez-Elisea et al., 1996). Similarly, relative humidity was controlled within a range of 60 to 70%. Notably, the greenhouse consistently provided a 10°C temperature advantage over outdoor conditions, highlighting the double-layer structure's effectiveness in fostering optimal vegetative growth.
The optimal temperature range for flowering induction following the vegetative growth period is from 5 to 15°C (Ramírez and Davenport, 2010). To achieve this in Korea, a heating and cooling system, including a thermal curtain and an electric fan, was implemented to create a suitable environment. During the flowering induction period (November 15, 2022, to January 15, 2023) the average temperature and relative humidity were maintained at 13.4°C and 62%, respectively, demonstrating effective environmental control. Altogether, utilizing a double-layer greenhouse and climate control (heating and cooling) system effectively established optimal conditions for both vegetative and reproductive growth in mangoes.
The GA inhibitor concentrations (PBZ 1,500 ppm and Pro-Ca 500 ppm) were selected based on previous research (Chusri et al., 2008; Pérez Barraza et al., 2016). A study in Okinawa, Japan, reported that applying PBZ 1,500 ppm twice per tree (each application being 1 liter), with a two-week interval, during the second vegetative growth period accelerated bud break by 18 days, initiated flowering five days earlier, and significantly increased the flower panicle percentage compared to control trees (Chusri et al., 2008). While the inflorescence length and thickness remained similar, the yield was significantly higher in the PBZ-treated group.
Another study examined the effects of Pro-Ca on ‘Ataulfo’ mangoes using various application dates and concentrations after harvest and pruning. The application of Pro-Ca at 500 mg·L-1 (at 30, 45, and 60 days after pruning) inhibited vegetative growth and advanced flowering by 20 days. A single Pro-Ca application of 1,500 mg·L-1 at 30 days after pruning advanced flowering by 35 days. These findings confirmed the efficacy of Pro-Ca in suppressing vegetative growth and promoting flowering (Pérez Barraza et al., 2016). Based on the evidence, PBZ 1,500 ppm and Pro-Ca 500 ppm were chosen for this experiment and applied after sufficient vegetative growth following pruning.
Vegetative growth, assessed by measuring the leaf length, leaf width, shoot length, and shoot thickness on October 21, 2022, revealed no significant differences between the control and PBZ and Pro-Ca treatments. These findings align with Nafees et al. (2010), who reported decreased vegetative growth at higher GA inhibitor concentrations. Given that the anti-GA concentrations used in this experiment were significantly lower than those in the cited literature, it is likely that they did not negatively impact vegetative growth development. Determining the optimal number and concentration of anti-GA treatments within a range that does not negatively affect vegetative growth can be done in further experimentation.
In the final stage of panicle development after full bloom, the growth of the reproductive organs was evaluated by measuring panicle length and width. Again, no significant differences were found between the control and any of the treatment groups on both parameters. While the GA inhibitors used in this experiment are known to block the GA biosynthesis pathway in plants, thereby inhibiting vegetative growth (Evans et al., 1999; Desta and Amare, 2021), cytokinin is the predominant hormone during mango reproductive growth (Davenport, 2007). Therefore, it is plausible that the GA inhibitors did not have significant effects on these parameters. Overall, our findings suggest that the different anti-GA treatments and concentrations employed in this experiment did not negatively impact either vegetative or reproductive growth in mango.
The present study investigated the impact of GA inhibitors on reproductive growth stages by comparing the dates at which Stages 2 (emerging bracts), 5 (elongated panicle), and 7 (full bloom) were reached. While no significant differences were observed between the control and Pro-Ca groups, the PBZ treatments accelerated early-stage development, with significantly earlier bract emergence (8 days) and panicle elongation (6 days). However, the advancement gradually narrowed during panicle development, and no significant differences were detected at full bloom in any treatment group. This finding aligns with observations by Geetha et al. (2016), who reported earlier flowering with increased initial temperatures. The observed temperature rise during panicle development might have attenuated the effects of PBZ on later stages.
The primary focus of this experiment was to assess flower bud differentiation rates, calculated as the ratio of panicles formed to potential panicle-bearing apical parts per tree. The control group exhibited 77.5% of differentiation rate, while the PBZ once treatment increased this to 94.5%. Notably, the PBZ twice, Pro-Ca once, and Pro-Ca twice treatments all achieved a 100% differentiation rate. While the PBZ once treatment did not differ significantly from the control, the remaining groups displayed significant increases in flower bud differentiation. These results validate the concentration and effectiveness of the chosen GA inhibitors in achieving the experiment’s objective: inducing flower bud differentiation.
Panicle morphology was categorized into four types: flower, leaf, mixed, and non-occurred. Interestingly, all treatment groups and the control group exhibited mixed panicles. The control exhibited a 22.5% occurrence of absent panicles, which was reduced to 5.5% in the PBZ once group. Although high nitrogen levels have been linked to mixed panicle formation (Ramírez and Davenport, 2010), the exact cause of this outcome remains unclear and warrants further investigation.
Finally, metabolite profiling was conducted via GC-MS on samples collected from the control and treatment groups at different time points. Eight common compounds were identified, consisting of one fatty acid (palmitic acid), one furfural metabolite (furfuryl alcohol), three phenolic compounds (syringol, pyrogallol, catechol), one antioxidant compound (DDMP), and two other compounds (benzoic acid, hydroxymethylfurfural). Notably, these compounds have been linked to roles in flower organ development and responses associated with flowering (Bhandari et al., 1998; Rademacher, 2000; Koeduka et al., 2009; Shen et al., 2019; Gawarecka and Ahn, 2021).
Furthermore, we identified four unique metabolites which exhibited stage-specific appearance and disappearance. The presence of 4,5-diamino-2-hydroxypyrimidine at Stage 1 suggests its potential involvement in terminating vegetative growth and initiating flowering. Similarly, the surge in dihydroconiferyl alcohol, phytol, and lupeol at Stage 4 hints at their potential roles in advancing the flowering process. However, our understanding of which metabolites directly influence mango flowering and how GA inhibitors impact the corresponding levels remains very limited. Further research is crucial to elucidate the specific roles of these compounds in controlling mango flowering and to investigate their biosynthetic pathways.
Conclusion
This study investigated the effects of the GA inhibitors PBZ and Pro-Ca on flowering bud differentiation and assessed changes in the GC-MS profile of the ‘Irwin’ mango. The results of assessments of vegetative growth and the full bloom stage suggested no significant differences between the control and both treatment groups, i.e., PBZ and Pro-Ca. Notably, this study found that GA inhibitors, specifically PBZ, accelerated early reproductive growth stages in mango plants. Further, the PBZ treatment induced earlier bract emergence and panicle elongation, but this progress narrowed during panicle development. Panicle development coincided with rising temperatures, revealing a diminishing effect during the initial reproductive growth stage. The experiment assessed the rate of flower bud differentiation, showing that the PBZ twice, Pro-Ca once, and Pro-Ca twice treatments achieved 100% differentiation. These results confirm the effectiveness of the GA inhibitors tested here. Furthermore, eight common metabolites were identified through metabolite profiling, all of which were associated with the flowering response. On the other hand, we found four unique stage-specific metabolites involved in advancing flowering processes. Overall, this investigation confirmed the positive effects of GA inhibitors in promoting flowering by controlling the vegetative growth of ‘Irwin’ mangoes. Further, the results here offer valuable insights for optimizing mango cultivation practices under controlled environments and could pave the way to overcoming the challenge of flower bud differentiation.
Supplementary Material
Supplementary materials are available at Horticultural Science and Technology website (https://www.hst-j.org).
- HORT_20240060_Table_S1.pdf
Supplementary Table S1. Comprehensively detected metabolites in mango ‘Irwin’
- HORT_20240060_Table_S2.pdf
Supplementary Table S2. Detected metabolites suddenly appear or disappear in mango ‘Irwin’