Introduction
Materials and Methods
Study area and experimental design
Sample collection
Determination of leaf and twig physiological indicators under cold stress
Statistical analysis
Results
Z. bungeanum leaf protective enzyme activity during the cold stress treatments
Leaf osmotic adjustment substances during the cold stress treatments
Moisture in leaves and branches during the cold stress treatments
Low temperatures affect MDA and REC in Z. bungeanum leaves
PCoA analysis of Z. bungeanum cultivars in response to cold stress treatments
Correlation and membership function evaluation
Discussion
Conclusion
Introduction
The plant Zanthoxylum bungeanum Maxim belongs to the Rutaceae family. Z. bungeanum is an important aromatic and medicinal plant; its peel has a unique flavor and provides a numbing sensation, and it is a popular cooking condiment as well (Hu et al., 2022). As a crop with high economic value, Z. bungeanum is rich in flavonoids and alkaloids, and it offers important protection value for human health (Fei et al., 2022). In addition, Z. bungeanum has several biological functions, specifically antibacterial, analgesic and anti-inflammatory functions, when used as medicine (Okagu et al., 2021). The volatile oil in Z. bungeanum has abundant antioxidant and can be used in cosmetics (Li et al., 2022). Its seeds are rich in fatty acids and also have the potential to produce biodiesel (Hou et al., 2019). However, temperature fluctuations during this mid-autumn period can easily harm Z. bungeanum and limit productivity the following year (Lei et al., 2016; Ihara et al., 2020). Low-temperatures are an uncontrollable factor affecting crop yields, and they may be the main source of stress, limiting the development of fruit trees, as well as a key cause of steep reductions in orchard yields (Fan et al., 2022). How do different Z. bungeanum cultivars respond to low-temperature stress in terms of their plant physiological and biochemical reactions? Knowing this is critical to understanding the tolerance of Z. bungeanum cultivars to cold stress conditions and maintaining high economical value.
Many plants develop frost tolerance when exposed to cold circumstances, enabling them to resist such temperatures via several levels of biochemical and morphological changes (Devireddy et al., 2021). Specifically, plants have several ways of resisting stress, such as forming more roots, reducing their leaf development, producing osmotic protective chemicals (Zhang et al., 2022), and shutting their stomata to safeguard the water content of their branches and leaves (Kwon et al., 2022). Plants can adjust antioxidant enzymes, osmotic regulators, and cell membrane permeability in response to cold stress conditions (Zhu, 2016; Pradhan et al., 2019). Low-temperature stress generally leads to increased membrane lipid peroxidation and ion leakage through reactive oxygen species (ROS) produced by the photosystem (Ozgur et al., 2013; Pradhan et al., 2019). As counter-defense against low-temperature stress, plants can rely on various processes physical (Balamurugan et al., 2018) and biochemical processes (Jeddi et al., 2021). The amount of intracellular ROS is maintained in a state of dynamic equilibrium by a series of non-enzymatic antioxidants and antioxidant enzymes (Tian et al., 2021). Cold stress is tolerated differently by different plant species, which rely on complex physiological and biochemical systems to adjust their metabolism and development accordingly. Being an apomictic plant, male flowers of Z. bungeanum have yet to be found for any cultivars (Tian et al., 2021). As a result, given that conventional hybridization makes it impossible to breed cold-resistant cultivars, it is necessary to screen for cultivars with high cold tolerance using physiological and biochemical criteria (Wassie et al., 2019). It was discovered that single shoots could be utilized to gauge plant development (Hua et al., 2015) and describe the phenology of vegetative growth (Yan et al., 2019). Because this method has few effects on plant growth, the cold-tolerance capabilities of plants can be assessed quickly.
This paper reports on the plant physiology of six Z. bungeanum cultivars under low-temperature stress. The dynamic changes in their physiological and biochemical substances were compared using chemometrics, and the important metabolic regulators were explained. Combined with a co-expression membership function analysis of each indicator, the pathway of each indicator participating in the tolerance response to low-temperatures was determined in an effort to explain the relationship between low-temperature stress and the cold tolerance of Z. bungeanum cultivars. This study can provide guidance to farmers and managers for exploring the response of Z. bungeanum and other closely related species to low-temperature stress conditions in agricultural settings.
Materials and Methods
Study area and experimental design
The testing site was located at the Zoucheng Modern Forestry and Fruit Science and Technology Research Institute (35°425'N, 117°104'E) in Jining City, Shandong Province, China, at an altitude of about 150 m. Here, a moderate temperate semi-humid continental monsoon climate prevails, with four distinct seasons, and rain and heat in the same period. The lowest average temperature in November reaches 0°C. The average minimum temperature in March-April is 0–5°C. Calcareous river alluvium, a major soil type in mountainous and hilly regions, is the parent material of the soil in this study area.
The experimental region was prepared for planting seedlings on formerly agricultural soil in March of 2017. The contents of the soil nutrients were as follows: total nitrogen at 0.75 g·kg-1, total phosphorus at 0.41 g·kg-1, total potassium at 18 g·kg-1, available nitrogen at 72 mg·kg-1, available phosphorus at 3 mg·kg-1, and available potassium at 79 mg·kg-1. Six Z. bungeanum cultivars — ‘Wucijiao’ (WC, from Hancheng), ‘Shizitou’ (SZ) and ‘Huanggai’ (HG) (from the Shaanxi Forestry Technology Extension Station and Z bungeanum Research Institute), ‘Shaocidahongpao’ (SC, from Shandong Agricultural University), and ‘Xinongwuci’ (XN) and ‘Gelaowuci’ (GL) (from Northwest A&F University) — were planted at the test site. In October of 2017, six Z. bungeanum cultivars were planted in the field. Each cultivar was planted in a rectangular field (50-m × 100-m plot).
Sample collection
Samples from each seedling were harvested in early November of 2020. Branches 20 cm in length with leaves on them were collected under identical management conditions; three replicates were used for each low-temperature (cold) stress treatment (including biological repetition). All leaves and branches of the plants were transferred to a full-strength Hoagland solution and placed in a growth chamber (BAGW-100, Hongda Boao Environmental Test Co., Ltd., China), where they were subjected to five cold stress treatments (‒4, ‒2, 0, 2, and 4°C; error ± 0.2°C). To avoid excessive instantaneous cooling, the experiment was conducted at a time-sharing rate of 4 C h-1, which was eventually lowered to the set cold stress level and then raised to room temperature at the same rate after 24 h. Leaves and stems of Z. bungeanum at the same position (i.e., opposite leaves at the top of branches and leaves) were cut for the determination of their physiological and biochemical indicators.
Determination of leaf and twig physiological indicators under cold stress
The superoxide dismutase (SOD) and peroxidase (POD) activities of Z. bungeanum sprouts were both measured by methods developed by Pan (Pan et al., 2020). The total soluble protein (SP), total soluble sugar (SS), proline (Pro) and malondialdehyde (MDA) levels were measured according to Pradhan (Pradhan et al., 2019). The levels of chlorophyll (Chl) were determined according to Wellburn (Wellburn, 1994). In order to measure the water content (WC) and relative conductivity (REC), the calculations followed the method outlined by Zhou et al. (2021) and Chu et al. (2016).
Statistical analysis
Statistical analyses of the branch/leaf physiological data and tests for significant differences in the means among the treatments (p < 0.05) were implemented in IBM SPSS v26.0 software (IBM Corp., Armonk, NY, USA). Origin (Origin Lab Corporation, v.2021) software was used to obtain box-plots. A principal coordinates analysis (PCoA) based on the Bray–Curtis distance was used to compare the differences in the physiological and biochemical indicators among the samples using the ‘vegan’ package (Dixon, 2003) in R v4.1.2 software (Werner et al., 2011). Indicator correlations were analyzed with the ‘corrplot’ package (Hahsler et al., 2008), with ‘desolve’ (Kneis et al., 2017) used for logistic equation modeling (Song et al., 2014). The fitted logistic equation is , for which LT50 = ln(a/b), and where y is the cell injury rate, is the temperature, K is the saturation capacity of the cell injury rate (K=100%), a and ‒b are equation parameters, and LT50 represents the semi-lethal temperature (Pingel, 2014). The membership function is calculated according to Liu (Liu et al., 2017).
In these equations, f (MCi) represents the membership function value of each main factor, and MCmax and MCmin respectively represent the maximum value and minimum value of the i-th comprehensive indicator value of Z. bungeanum. When there is a positive correlation between a certain indicator and the cold-tolerance coefficient, it is calculated by formula (1); conversely, when the correlation is negative, it is calculated by formula (2).
Results
Z. bungeanum leaf protective enzyme activity during the cold stress treatments
The POD contents and SOD activities of six Z. bungeanum cultivars exhibited a similar pattern of dynamic change: first increasing and then decreasing under differing low-temperatures (Fig. 1A). The POD content of the GL, SZ, XN, and HG cultivars was highest at 2°C, whereas that of both the SC and WC cultivars was highest at 0°C. The SC had the highest POD activity, reaching a maximum of 676.67 U·g-1 FW (fresh weight) at 0°C, and its POD activity was greater than that of other Z. bungeanum cultivars under low-temperature stress. The rise in GL was smallest among the cultivars when exposed to 2°C, being 18.5 U·g-1 FW. When compared to 4°C, the maximum and lowest SOD activity levels were correspondingly 488.94 U·g-1 FW in HG at 2°C (p < 0.01) and 78.02 U·g-1 FW in SC at ‒4°C (Fig. 1B). As the temperature decreased, the SOD activity of the six Z. bungeanum cultivars generally followed a sigmoidal response curve, rising dramatically at 2°C, with WC, SC, and HG rising by 192.76%, 88.88%, and 127.57%, respectively. The SOD activity of WC and SC peaked at 0°C, at 361.72 and 398.97 U·g-1 FW, while that of HG, XN, SZ, and GL peaked at 2°C, being 488.94, 309.48, 341.79, and 334.57 U·g-1 FW, respectively. After 2°C, the SOD activity of Z. bungeanum had essentially surpassed the activity threshold and began to decline.
Leaf osmotic adjustment substances during the cold stress treatments
This study explored the cellular responses to osmotic damage, where the SP, SS, Pro, and Chl contents of the leaves were determined (Figs. 1C, 1D, and 2). The WC and SZ cultivars showed the greatest increase, at 53.02% and 83.00%, respectively, in the low-temperature range of 4–2°C. Except for SC, the SP concentration of the Z. bungeanum cultivars increased in the colder range of ‒2 ~ ‒4°C. The highest SS values for SZ and HG were 2°C, at 0.36 and 0.29 µg·g-1, respectively. The highest SS outcomes for the SC, GL, and WC cultivars at 0°C were 0.35, 0.28, and 0.28 µg·g-1, respectively, but for XN, its maximal SS value (0.35 µg·g-1) was attained at 2°C.
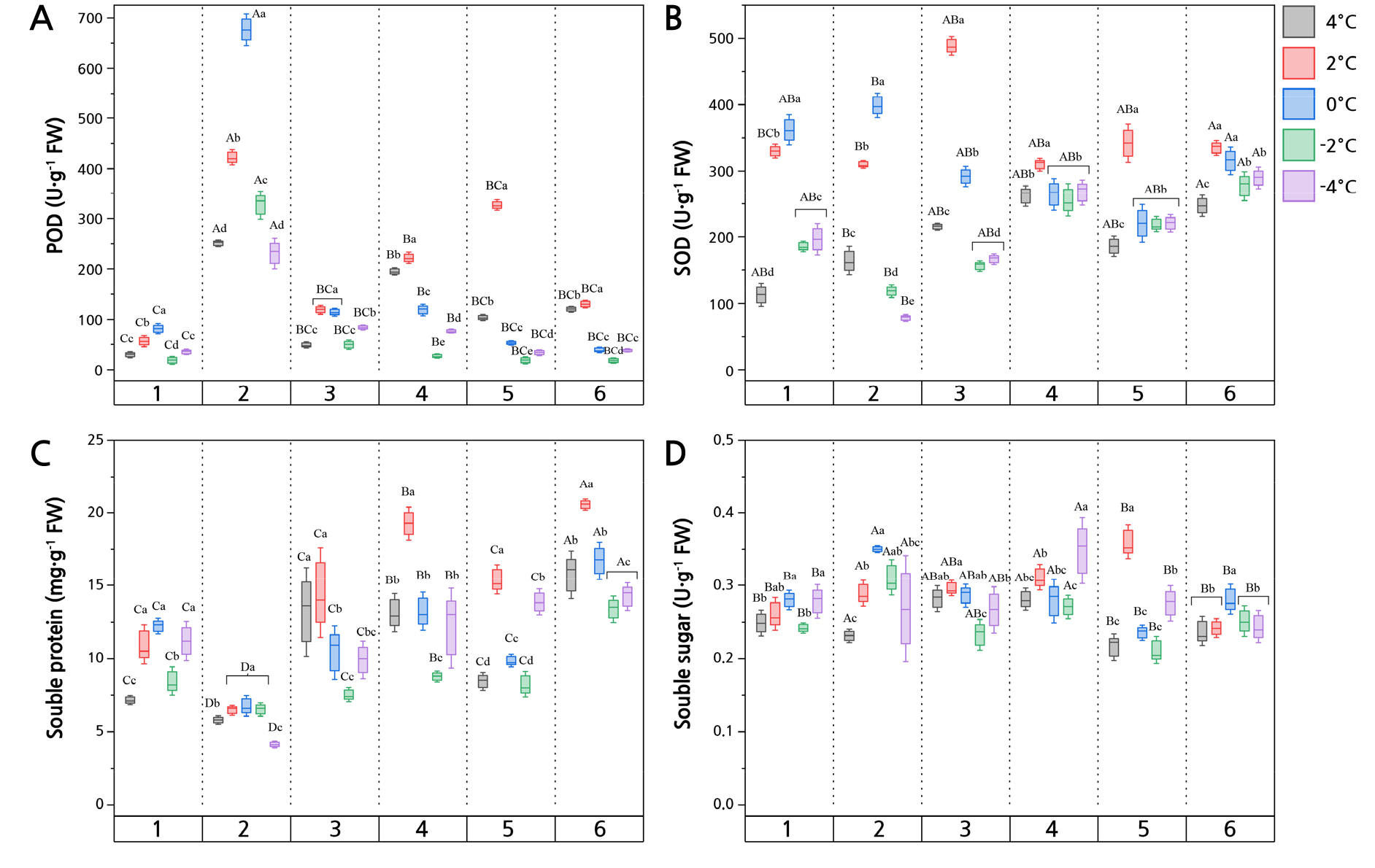
Fig. 1.
Protective enzymes activity and soluble substances in leaves of Z. bungeanum exposed to different low-temperature (cold) stress treatments (data shown are the mean ± SD). Based on Duncan's one-way ANOVA, different uppercase letters indicate significant differences among the cultivars, and different lowercase letters indicate significant differences among the temperatures (p < 0.05). 1, Z. bungeanum ‘Wucijiao’; 2, Z. bungeanum ‘Shaocidahongpao’; 3, Z. bungeanum ‘Huanggai’; 4, Z. bungeanum ‘Xinongwuci’; 5, Z. bungeanum ‘Shizitou’; 6, Z. bungeanum ‘Gelaowuci’. The same as below.
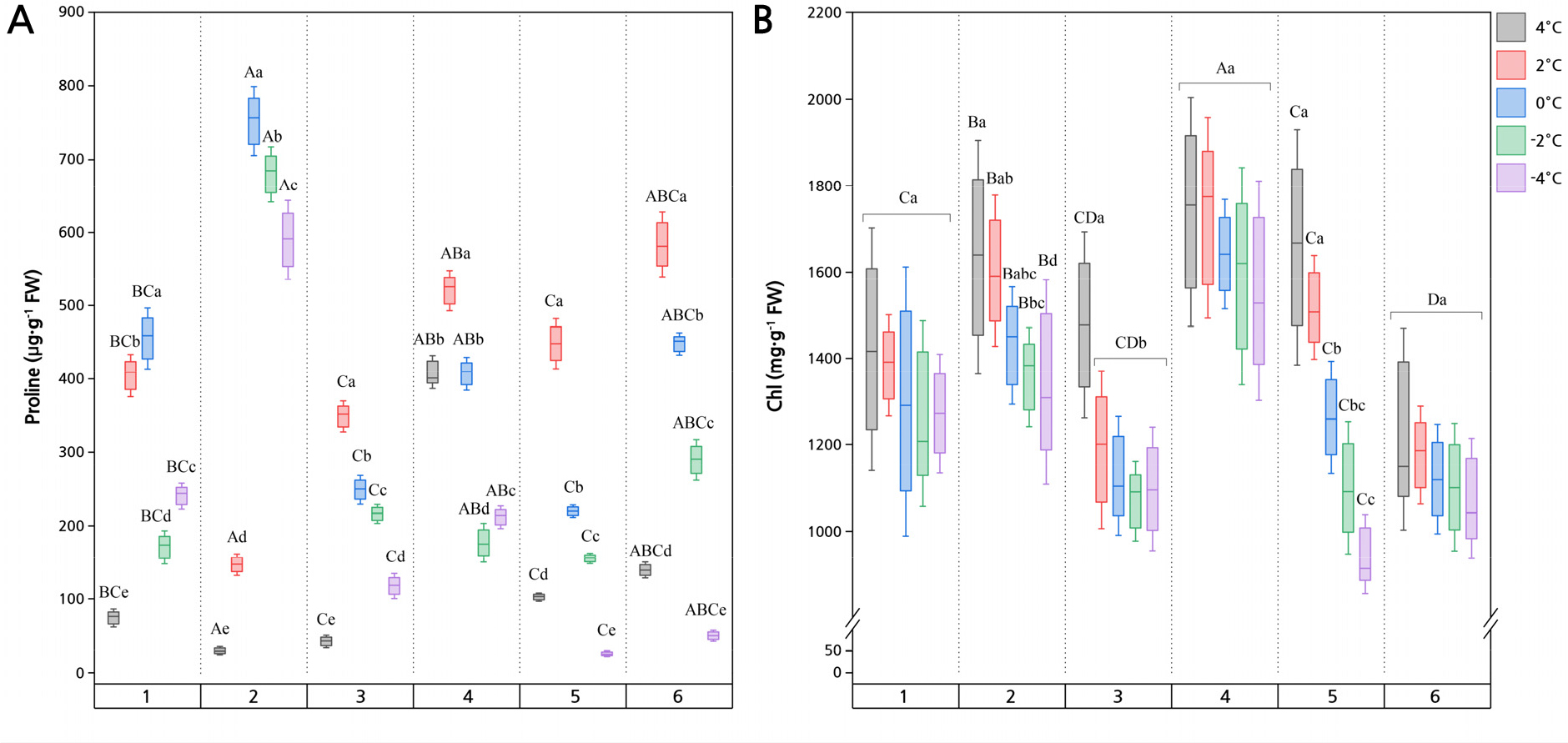
Fig. 2.
Pro and Chl contents in Z. bungeanum leaves exposed to different low-temperature (cold) stress treatments. Different uppercase letters indicate significant differences among the six cultivars, and different lowercase letters indicate significant differences among the processing temperatures (p < 0.05).
The Chl and Pro contents of the Z. bungeanum cultivars were significantly affected by the temperature (Fig. 2; p < 0.05). The XN cultivar had the greatest mean Chl content when compared with the other Z. bungeanum cultivars. The Pro contents of the GL, SZ, XN, and HG cultivars reached their maximum values of 583.83, 448.17, 520.48, and 348.48 µg·g-1 at 2°C, respectively, whereas SC and WC reached their maximum values of 752.23 and 455.15 µg·g-1 at 0°C.
Moisture in leaves and branches during the cold stress treatments
Lower temperatures are a major reason for the reduced moisture content of Z. bungeanum leaves and branches (Fig. 3A and 3B). The moisture contents of the SC and SZ branches were 43.53% and 42.37% at 4°C, respectively. However, there was no significant difference in the GL value between the cultivars and low temperatures (p > 0.05). The corresponding moisture contents in the SC and HG leaves were 38.08% and 38.77% at ‒4°C, which still exceeded those in the other cultivars (Fig. 3B). HG and SZ were not significantly affected by the temperature, nor did they differ between the leaves and branches.
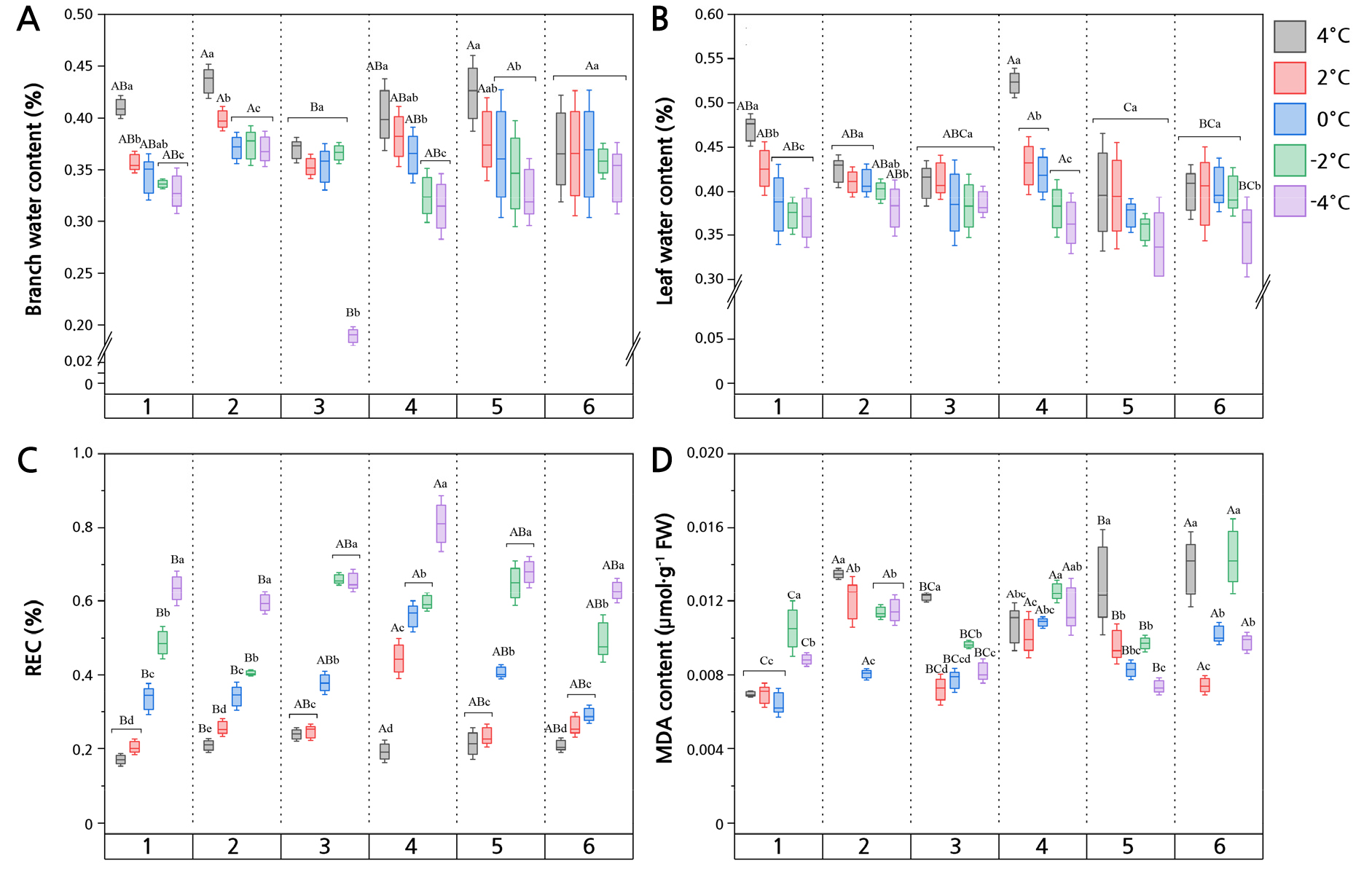
Fig. 3.
REC and MDA of Z. bungeanum exposed to different low-temperature (cold) stress treatments. REC: relative conductivity; MDA: malondialdehyde. Different uppercase letters indicate significant differences among the cultivars, and different lowercase letters indicate significant differences among the temperatures (p < 0.05).
Low temperatures affect MDA and REC in Z. bungeanum leaves
The leaf MDA and REC outcomes varied significantly depending on the cultivar and temperature (Fig. 3C and 3D). The highest MDA content was found at ‒2°C for GL and the lowest was at 0°C for WC, with corresponding values of 0.014 and 0.007 µmol·g-1 FW. In addition, SZ, SC, and HG had the highest MDA contents at 4°C. The REC value of Z. bungeanum leaves tended to increase with a decrease in the temperature (Fig. 3D). All six cultivars showed the highest REC at ‒4°C, with the corresponding values being 63.49% (Z. bungeanum ‘Wucijiao’), 59.51% (Z. bungeanum ‘Shaocidahongpao’), 64.34% (Z. bungeanum ‘Huanggai’), 81.10% (Z. bungeanum ‘Xinongwuci’), 67.93% (Z. bungeanum ‘Shizitou’), and 62.93% (Z. bungeanum ‘Gelaowuci’) (Fig. 3C). Interestingly, by fitting the logistic equation (Fig. 4), LT50 led to good sigmoidal curves fitted between 4°C and ‒4°C, all having R2-values exceeding 0.90 (i.e., more than 90% of the variation explained by the model). There were pronounced differences in the semi-lethality temperatures of the Z. bungeanum cultivars. For example, SZ reached a semi-lethal state at 1.2°C, whereas GL only did at ‒12.7°C.
PCoA analysis of Z. bungeanum cultivars in response to cold stress treatments
Based on the first two components, PC1 (74.95%) and PC2 (19.89%), it was possible to distinguish the Z. bungeanum cultivars from each other (Fig. 5A). There were less variations among the WC, HG, XN, and SZ cultivars, which clustered in ordinal space; in contrast, the SC samples showed significant sample separation and interspecific heterogeneity. Regarding low-temperature stress, there is a significant difference among the cultivars between 4°C and 2°C and yet no significant difference between ‒2°C and ‒4°C (Fig. 5B). The physical indicators (such as LWC, BWC and REC) were closely related to the low-temperature, while the other biochemical indicators showed inhomogeneous linear correlations (Suppl. Fig. 1).
Correlation and membership function evaluation
The heatmap showed a negative correlation between the enzyme activity and MDA level under low-temperature stress (Fig. 6). The average weights of all physiological indicators of Z. bungeanum were close to 0.1, which indicates a close correlation with cold resistance. Among them, SZ had the lowest overall evaluation value (0.377), indicating its poor cold resistance (Table 1).
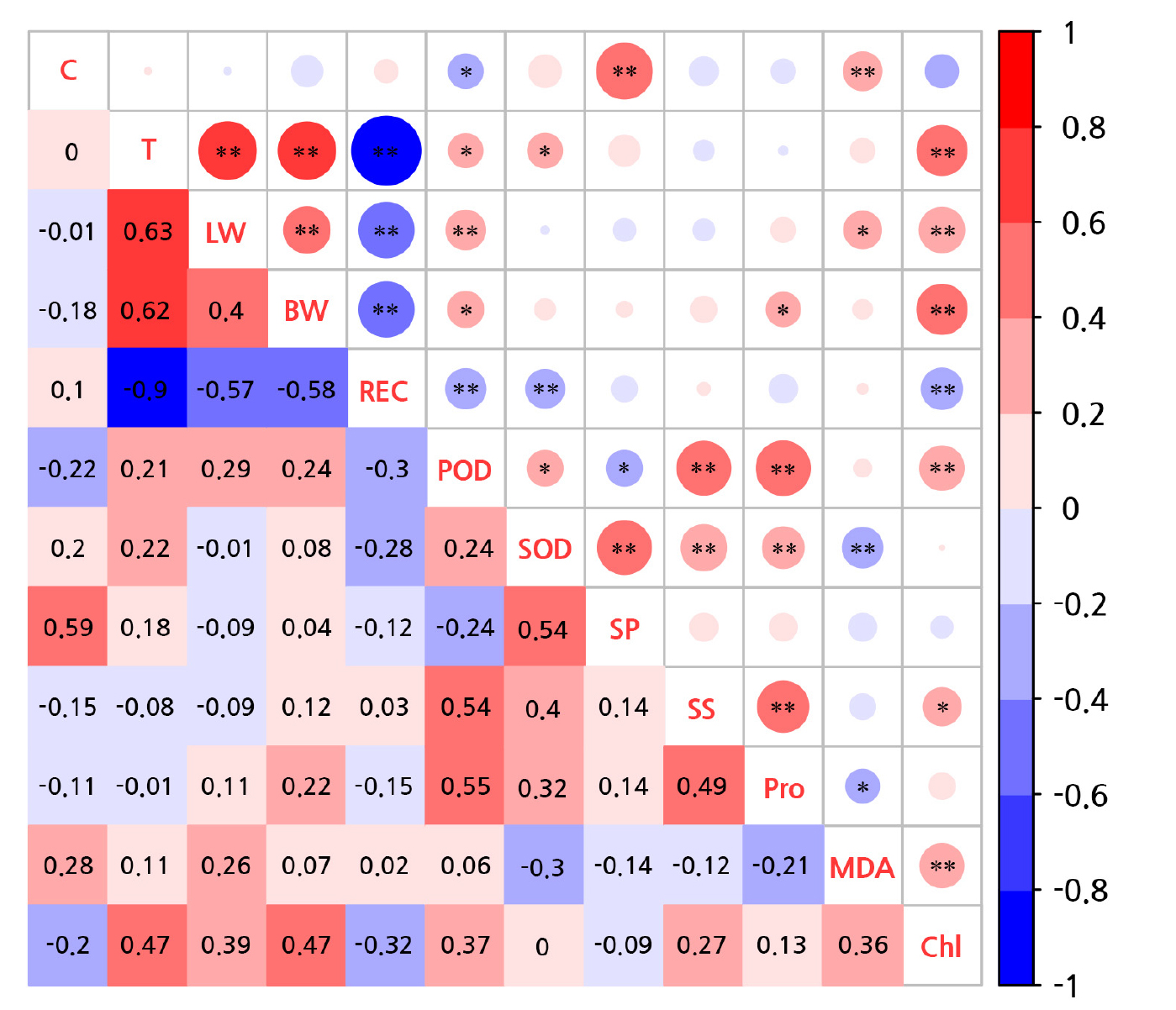
Fig. 6.
Correlations between Z. bungeanum physiological and biochemical indicators and low temperatures. Two experimental treatments: C, cultivar; T, temperature. Ten physiological indicators: LW, leaf water content; BW, branch water content; REC, relative electric conductivity; SOD, superoxide dismutase; POD, peroxidase; SP, soluble protein; SS, soluble sugar; Pro, proline; MDA, malondialdehyde; Chl, chlorophyll. There were at least three replicates of each indicator. *p < 0.05.; **p < 0.01.
Table 1.
Comprehensive Evaluation of the Cold Resistance of Different Z. bungeanum Cultivars
Index\cultivar | WC | SC | HG | XN | SZ | GL | |||||||
Value | Weight | Value | Weight | Value | Weight | Value | Weight | Value | Weight | Value | Weight |
Average weight | |
LWC % | 0.775 | 0.151 | 1.000 | 0.148 | 0.000 | 0.138 | 0.692 | 0.108 | 0.770 | 0.141 | 0.843 | 0.107 | 0.132 |
BWC % | 0.628 | 0.152 | 0.857 | 0.136 | 1.000 | 0.123 | 0.503 | 0.122 | 0.000 | 0.129 | 0.181 | 0.107 | 0.128 |
REC % | 0.185 | 0.052 | 0.000 | 0.067 | 0.285 | 0.058 | 1.000 | 0.030 | 0.390 | 0.051 | 0.155 | 0.052 | 0.052 |
POD U·g-1 | 0.008 | 0.077 | 1.000 | 0.078 | 0.252 | 0.064 | 0.216 | 0.162 | 0.000 | 0.113 | 0.023 | 0.218 | 0.119 |
SOD U·g-1 | 0.559 | 0.053 | 0.000 | 0.093 | 0.418 | 0.092 | 0.894 | 0.088 | 0.670 | 0.088 | 1.000 | 0.084 | 0.083 |
SP µg·g-1 | 0.700 | 0.084 | 0.000 | 0.125 | 0.571 | 0.147 | 0.789 | 0.091 | 0.964 | 0.084 | 1.000 | 0.100 | 0.105 |
SS µg·g-1 | 0.329 | 0.118 | 0.240 | 0.097 | 0.219 | 0.123 | 1.000 | 0.086 | 0.307 | 0.094 | 0.000 | 0.097 | 0.103 |
Pro µg·g-1 | 0.381 | 0.029 | 1.000 | 0.007 | 0.163 | 0.021 | 0.330 | 0.115 | 0.000 | 0.057 | 0.043 | 0.042 | 0.045 |
MDA µmol·g-1 | 0.663 | 0.148 | 0.046 | 0.102 | 0.808 | 0.079 | 0.000 | 0.099 | 1.000 | 0.079 | 0.455 | 0.079 | 0.098 |
Chl mg·g-1 | 0.534 | 0.137 | 0.654 | 0.147 | 0.247 | 0.155 | 1.000 | 0.099 | 0.000 | 0.162 | 0.211 | 0.114 | 0.136 |
Comprehensive evaluation | 0.531 | 0.473 | 0.410 | 0.575 | 0.377 | 0.369 | |||||||
Order | 2 | 3 | 4 | 1 | 5 | 6 |
Discussion
The activity of plant defensive enzymes fluctuates in response to adverse stress conditions, with the ultimate objective being to restore cellular stability. Lower temperatures can cause the generation of reactive oxygen species (ROS) within plants, which can harm their growth and development (Chowdhury et al., 2015). This arises because low-temperature stress can lead to greater lipid peroxidation of the cell membrane (Biswas et al., 2012; Hashem et al., 2018). As components of the antioxidant enzyme system, SOD and POD can remove ROS and prevent oxidative stress from harming plants (Gulen et al., 2008). SOD converts O-2 to H2O2, whereas POD further degrades H2O2 into H2O and O2, hence minimizing low-temperature damage (Roychoudhury et al., 2012). MDA is the main product of membrane lipid peroxidation, and its concentration is usually directly correlated with the degree of membrane peroxidation (Xu et al., 2021). The results showed that the activity of antioxidant enzymes increased during the initial stage of low-temperature stress, indicating that the cold stress response mechanism had begun by this time in Z. bungeanum. The POD and SOD activities of both the WC and SC cultivars increased in the range of 4–0°C but decreased considerably thereafter. Meanwhile, the MDA content was negatively correlated with the SOD activity. This suggests that the more augmented the protective enzyme activity is, the greater the cold tolerance of the plant also is, and that its capacity to scavenge ROS would gradually improve. However, the bolstered protective enzyme activity has a limit. The increased MDA buildup will disrupt the normal expression of the protective enzyme activity and diminish its positive defensive function, exacerbating plant body damage caused by an unrestrained reaction to ROS (Ellouzi et al., 2011). Additionally, the protective enzyme activity of Z. bungeanum with strong stress tolerance is maintained at a lower level within a certain temperature range, but it increases to a higher level when the plant is subjected to adverse conditions, which ensures that once the chilling injury ends, it may resume normal growth. The protective enzyme activity of those Z. bungeanum cultivars (i.e., XN, WC) showing cold-hardiness is obviously expressed, as these cultivars are highly able to remove harmful substances such as MDA, or MDA produced by the low-temperature stress itself is lessened. Combined with the results of the present study, we suggest that the strategies of different Z. bungeanum cultivars coping with low-temperatures are similar and that the antioxidant system plays crucial roles in the response to cold stress.
Plants can maintain their osmotic balance and cell membrane integrity by regulating osmotic adjustment substances (Pro, SP, SS) (Yan et al., 2019), which can temporarily lower the internal freezing point, which this process serving as a protective mechanism against adversity (El Moukhtari et al., 2020; Hinai et al., 2022). At the onset of low-temperature stress, the levels of SS, SP, and Pro in Z. bungeanum leaves tend to rise as the temperature drops (Figs. 1C, 1D, and 2A). The results showed that carbohydrates not only offer an adequate carbon source for plants but also activate other enzymatic responses and speed up the physiological process of coping with cold (Wang et al., 2018). Low-temperature stress induces the accumulation of solutes such as SP and SS in Z. bungeanum, increasing the overall solute concentration, which can prevent the cytoplasm from forming small ice crystals. Previous studies have shown that plants increase their SS activity and decrease their MDA content as the temperature decreases (Tian et al., 2021; Hu et al., 2022), suggesting that SS, SP and Pro can improve cold tolerance in Z. bungeanum. Furthermore, the changes to Pro and SP in the WC, SC, and XN cultivars are comparable. The Pro content increased significantly at 4–2°C but decreased slowly at 2°C ~ ‒4°C. This may be related to the fact that Pro can induce the expression of SP, which is sensitive to low-temperature stress, so as to improve the adaptability of plants to low temperatures (Ben Rejeb et al., 2014; Hinai et al., 2022). The intracellular environment can be kept reasonably steady via the protective impact from osmotic adjustment chemicals on cell membrane lipids (Cao et al., 2012), enabling plants to guard against low-temperature stress to the greatest degree possible. The Pro contents of both the SC and WC cultivars reached their maximum at 0°C, indicating that as the temperature dropped, the intracellular water retention capacity would decrease, making it difficult to augment the contents of internal organic matter and inorganic salts (Banakar et al., 2022). This also suggests that cold stress causes relatively more damage to cell membranes and worsens the overall mechanical damage incurred by Z. bungeanum plants. Chl has an important function in the cold response in terms of cell integrity, as it preserves membrane permeability, boosts carbohydrate and protein synthesis, and raises the cell sap content (Sawicki et al., 2015). However, under low-temperature stress, the photosynthetic rate of plants will arguably decrease, which directly affects the structure and activity of the photosynthetic tissues (Panda et al., 2020), resulting in greater cell electrolyte extravasation and reduced photosynthesis (da Silva et al., 2021). Cold stress will restrict Chl synthesis (Kalisz et al., 2016), limiting plant development and thus directly affecting the photosynthetic potential (Chen et al., 2016). An adequate Chl content not only ensures a proper photosynthetic rate and is required for robust respiration, but it also promotes the synthesis of SS, SP, and other solutes and in this way regulates cell osmosis (El-Sabagh et al., 2019). The Chl content of Z. bungeanum dropped as the temperature fell in the experiment, perhaps due to the enhanced Chl enzyme activity brought on by the cold stress, which hastened the breakdown of the Chl enzyme (Huang et al., 2017).
Due to their exposure to generally low-temperatures, the water content of the Z. bungeanum branches and leaves dropped, indicating that their protective effects, including their enzymatic activity, could not endure adverse stress and could not sustain the normal respiratory demand of Z. bungeanum leaves (Gong et al., 2020). Linear correlations indicated that physical indicators (i.e., LWC, BWC and REC) were consistently related to low-temperatures in each case (Suppl. Fig. 3), whereas, among other physiological indicators (such as Pro in the Z. bungeanum WC cultivar), enzyme activities and osmoregulatory substances were negatively correlated with reduced temperatures in several Z. bungeanum cultivars, suggesting that plants rely on multiple factors to cope with low-temperature stress. The logistic modeling results showed that the semi-lethal temperature of different Z. bungeanum cultivars varied substantially; GL reached a near-death state at ‒12.7°C, whereas XN was affected at 1.2°C. This disparity is likely linked to the dehydration and destruction of cell structures in plants under low-temperature stress, considering that it is imperative to retain the integrity of their cell structures under stressful conditions (Ding et al., 2019). For the branches of SC and the leaves of XN, the water content remained at a high level despite the low-temperatures. Furthermore, although plants are damaged by exposure to low-temperature stress, transpiration of their branch and leaf tissues can continue, resulting in water loss and weakened plant tolerance to cold stress and perhaps subsequent stress factors (Wang et al., 2021).
Logistic modeling showed that the low-temperature semi-lethal temperature LT50 of leaf conductivity was from ‒12.7 °C to 1.2°C. The logistic model analysis indicated that the LT50 order for the six Z. bungeanum cultivars was as follows: ‘Gelaowuci’ > ‘Shaocidahongpao’ > ‘Wucijiao’ > ‘Huanggai’ > ‘Shizitou’ > ‘Xinongwuci’. According to the analysis of the membership function, the cold tolerance of the six Z. bungeanum cultivars was in the following order: ‘Xinongwuci’ > ‘Wucijiao’ > ‘Shaocidahongpao’ > ‘Huanggai’ > ‘Shizitou’ > ‘Gelaowuci’. ‘Gelaowuci’ was the strongest according to LT50, while ‘Xinongwuci’ was the most resistant according to the physiological indicators by membership function. In summary, the threshold of plant resistance to semi-lethal low-temperatures can be predicted based on relative conductivity of the leaves. The membership function analysis revealed that physiological indices can be among the parameters used to assess cold resistance in Z. bungeanum. With regard to production management, these criteria can be used to verify the cold resistance of Z. bungeanum, with emphasis on resolving the important physiological and biochemical functions of antioxidant enzymes during adverse stress. For those cultivars with weak cold resistance, Z. bungeanum can adapt to a cold environment by releasing smoke and smearing calcium polysulfide in November. This preventative approach is also beneficial for the regular growth of Z. bungeanum the following March and April. Consequently, predicting cold-resistant Z. bungeanum cultivars using reasonable physiological and biochemical indicators can lead to better improvements in the agricultural production of these and similar Z. bungeanum plants.
Conclusion
The REC outcome of Z. bungeanum increased with the aggravation of low-temperature stress in this experiment. The levels of SS and Pro showed a significant positive association, whereas POD, SOD, and REC showed significant negative correlations (p < 0.05). The first stage of physiological stress in five Z. bungeanum cultivars was identical, although PCoA differed according to the temperature and cultivar. In summary, a membership function analysis revealed that XN and WC could retain their growth dominance at later stages of low-temperature stress, indicating they are relatively cold-resistant types. Therefore, it is possible to select cold-resistant cultivars of Z. bungeanum based on their physiological and biochemical characteristics, which are directly useful for the agricultural production of this plant.
Supplementary Material
Supplementary materials are available at Horticultural Science and Technology website (https://www.hst-j.org).
- HORT_20230044_Figure_1s.pdf
Relationships between physiology indexes and cold stress of six Zanthoxylum bungeanum cultivars. The shadow indicates the 95% confidence interval. A linear equation was used to fit the regression equation. Only cases with statistically significant fitted lines (p < 0.05) are shown.