Introduction
Materials and Methods
Plant Materials and Environmental Conditions
Root-zone Cooling Systems
Electrolyte Leakage Analysis
Photosynthetic Trait
Chlorophyll Fluorescence
Growth Characteristics
Data Collection and Statistical Analysis
Results
Medium Temperature Changes and Root Electrolyte Leakage
Photosynthetic Traits of the Paprika Transplants
Chlorophyll Fluorescence of the Paprika Transplants
Growth of the Paprika Transplants
Discussion
Introduction
Heat stress affects the productivity of the field- and greenhouse-cultivated plants. Paprika (Capsicum annuum L.) plants are originally from tropical regions and require air temperatures of less than 32°C for optimal growth and development (Mercado et al., 1997). High air temperatures above 32°C decrease plant heights and induce axillary bud formation (Rylski and Spigelman, 1986) and the abscission of flowers and buds (Aloni et al., 1994; Saha et al., 2010), reducing the fruit set (Khah and Passam, 1992; Aloni et al., 2001; Erickson and Markhart, 2002) and seed development (Bakker, 1989; Aloni et al., 2001) of paprika. Heat stress also negatively affects the leaf photosynthesis (Bhandari et al., 2018) and water potential (Choi et al., 2013, 2014) of paprika plants before and after transplanting.
High air temperatures straightforwardly increase the root-zone temperature (RZT) given that hydroponically grown paprika plants before and after transplanting in greenhouses have limited root-volumes, such as plug trays and slabs, respectively. As the RZT increase, oxygen levels with root respiration decrease (Ityel et al., 2014) and root activity decrease (Choi et al., 2014). Paprika plant growth is promoted when the RZT is increased to 30°C, but the leaf areas and dry weights both decrease at a RZT of 36°C (Gossellin and Trudel, 1986). When both shoots and roots are heat-stressed, roots may often be more sensitive to heat stress than shoots in plants (Huang et al., 2012; Heckathorn et al., 2013), especially in paprika plants (Rylski, 1972) due to less heat-related protein synthesis taking place in roots than in leaves (Aloni et al., 1992). Because root development greatly affects the growth of seedlings as well as growth after transplanting (Grossnickle, 2005; Grossnickle and Ivetić, 2022), RZT management is crucial for the growth and development of the whole paprika plant.
Temperature management in greenhouses is particularly difficult due to high air temperatures in the summer seasons (Willits and Peet, 2001). Cooling methods in greenhouses, such as forced ventilation, fan and pad, fogging, and shading, are being applied to alleviate the adverse effects of heat stress (Willits, 2000). However, maintaining a cool temperature for greenhouses overall consumes much energy and is not cost-effective or environmentally friendly (An et al., 2021). Plant organ-based or local ambient cooling systems appear promising as specific technical solutions to increase energy efficiency rates. With these cooling systems, it is unnecessary to cool the entire greenhouse as plant damage can be mitigated to a certain extent if the RZT can be maintained within a normal range under high air temperatures (Kuroyanagi and Paulsen, 1988; Sasaki and Itagi, 1989). Among organ-based local cooling systems, root-zone cooling (RZC) can serve as a supplemental or an alternative system for hydroponic cultures of paprika in greenhouses during the summer with improved energy efficiency and root development.
There are different methods through which RZC can be applied. Air ducts (Choi et al., 2013) and water circulation (Won et al., 2007; Choi et al., 2014; Kwack et al., 2014) using a system of cooling pipes constructed into or adjacent to the growing media have been studied. It is also possible to cool the medium directly by chilling the nutrient solution as it is supplied to the plants. Each method has advantages and disadvantages in terms of practical application. However, the effectiveness of different RZC methods on the physiological and morphological traits of paprika has not been studied appropriately. To promote paprika transplant growth during the summer season in a greenhouse, the effects of different RZC methods (specifically, nutrient solution and water circulation cooling) on the photosynthetic traits and growth of paprika transplants were investigated under a condition that mimicked high mid-summer air temperature patterns using growth chambers with proper environmental control strategies.
Materials and Methods
Plant Materials and Environmental Conditions
Paprika (Capsicum annuum L. cv. Scirocco) seeds were sown in rockwool plugs (diameter × height = ø 20 × 27 mm, Grodan Co., Ltd., Netherlands), covered with vermiculite, soaked in a standard nutrient solution for paprika consisting of NO3-N 15.58, NH4-N 0.74, H2PO4-P 1.25, K 6.6, Ca 4.84, Mg 1.72, and SO4-S 1.72 mM for macronutrients, and Fe-EDTA 35, Mn 18.5, B 60, Cu 0.9, Mo 0.8, and Zn 8.5 mM for micronutrients (EC 2.2, pH 5.5) and then placed in an incubator maintained at an air temperature of 27°C for five days. When the seeds germinated, they were moved to growth chambers and grown for 20 days under the following environmental conditions: warm-white LEDs (Model v3.0_B, Futuregreen Co., Ltd., Suwon, South Korea) (Fig. 1) with a photosynthetic photon flux density (PPFD) and a photoperiod of 250 µmol·m-2·s-1 and 14 h·d-1 (from 5 am to 7 pm), respectively. Using the bending transplanting technique, seedlings were transplanted to 1.4 L white pots filled with coir substrate (crushed chips: dust = 50: 50; Power, Daeyoung GS Co., South Korea) and grown for 28 days. During this time, the daily air temperature was set in a pattern of four periods mimicking the change in the summer season’s air temperature: 26°C from 5 am to 11 am, 39°C from 11 am to 4 pm, 26°C from 4 pm to 7 pm, and 24°C from 7 pm to 5 am of the next day, designated the before heating period (BHP), heating period (HP), after a heating period (AHP), and dark period (DP), respectively. The temperature pattern was designed based on actual air temperatures measured at Suwon, South Korea, in July of 2021. Air temperatures were maintained by timer-controlled fans and with a temperature controller connected to a heater for each chamber, in conjunction with an air conditioner for the entire growth room. The air temperature and relative humidity in the chambers were determined using thermorecorders (TR-72wb, T&D Co., Japan) (Fig. 2). The corresponding relative humidity measured during the BHP, HP, AHP, and DP periods were 75.4, 50.5, 79.1, and 88%, respectively. The transplants were irrigated with the standard nutrient solution for paprika (EC 2.2, pH 5.5) by drip irrigation for 3 min (equivalent to 100 mL of nutrient solution per pot) every hour from 11 am to 4 pm. The RZT was measured every ten seconds with thermocouples at two positions (center and side points). The water temperature in the outlet and inlet of the silicone pipes and the nutrient solution temperature were measured every 15 seconds and recorded using a data logger with Tapaculo Lite software (UA11-K, Radionode Co., Yongin, South Korea).
Root-zone Cooling Systems
The experiment involved three treatments: No-cooling, nutrient solution cooling (NSC), and water circulation cooling (WCC) for RZC. Each treatment group contained eight plants and was replicated three times. In the No-cooling treatment, the plants did not receive root cooling, and the nutrient solution was maintained at 25°C when supplied. In the NSC treatment, the nutrient solution was chilled to 15.9 ± 0.8°C on average with a water chilling machine (DA-500B, Daeil Co., Korea) before being irrigated onto the growing media via drippers (3 min, equivalent to 100 mL of nutrient solution per pot, every hour during the HP, as stated above). The energy usage per plant for the NSC treatment, calculated via the multiplication of the amount of nutrient solution supplied to each plant per treatment per day (5 times × 100 mL = 0.5 L), the reduction in the nutrient solution temperature (∆T = 25–15.9 = 9.1°C ), and the water-specific heat capacity (1 kcal· kg-1 °C-1), was 4.55 kcal·d-1 per plant. In the WCC treatment, silicone pipes (1 cm diameter) were coiled four times in the pot around the growing media, and the water temperature was maintained at 15.9 ± 0.8°C; the water was circulated with submerged electric pumps through these pipes during the HP. The flow rate of this water for each chamber of eight plants was adjusted to 45 L·min-1 so that the water temperature at the outlets of these pipes could be maintained at 17.2 ± 1.0°C. The energy usage for the WCC treatment, calculated via the multiplication of the flow rate of water through each chamber (45 L·min-1 or 2,700 L·h-1), the duration of cooling (5 hours, from 11 am to 4 pm), the temperature difference between the inlet and outlet (∆T = 17.2–15.9 = 1.3°C), and water-specific heat capacity (1 kcal·kg-1 °C-1), divided by eight plants, was 2,194 kcal·d-1 per plant.
Electrolyte Leakage Analysis
Electrolyte leakage (EL) levels were measured as described by Lee et al. (2012) and modified slightly for roots. After the high-air-temperature treatment, the root tips were detached and washed with distilled water to remove the substrates. The samples were placed into 50 mL conical tubes containing 20 mL of triple-distilled water. At room air temperature, the tubes were shaken at 125 rpm on a shaker for 24 h and the electrical conductivity (EC) was measured by an EC meter (HI991300, Hanna Instruments, Woonsocket, RI, US). After autoclaving the sample at 120°C for 30 min, EC was measured again. The % EL value was calculated as follows (Arora et al., 1992):
Photosynthetic Trait
Three transplants from each treatment were randomly selected and photosynthetic traits were measured on a single 4th to 5th and 7th to 9th true leaf from the cotyledon at 13 and 27 DACT, respectively, with a portable photosynthesis system (LI-6800; LI-COR) equipped with a multiphase flash fluorometer. The leaves were exposed to light at the same red (R), blue (B), and far-red (FR) light ratios (R: B: FR=74: 12: 14) in the chamber with different light intensity levels from 0 to 2,000 µmol·m-2·s-1 to construct light response curves for the CO2 assimilation rate (An), stomatal conductance (Gs), and transpiration rate (E). Using the same leaves, two light response curves were constructed for each treatment at BHP and HP. The air temperature and relative humidity of the LI-6800 chamber at BHP and HP were set to 27°C and 55% and 40°C and 45%, respectively. Common settings for the chambers were as follows: a chamber flow of 400 µmol·s-1, chamber overpressure of 0.2 kPa, a fan speed of 10,000 rpm, and a CO2 concentration rate of 800 µmol·mol-1.
Chlorophyll Fluorescence
The 4th to 5th and 7th to 9th expanded leaves from the cotyledon were selected. The chlorophyll fluorescence parameters of the paprika transplants leaves were measured using the LI-6800 photosynthesis measurement system with multiphase flash fluorescence (6800-01) at 14 and 28 DACT. To avoid CO2 concentration fluctuations in the chamber, a CO2 cartridge was connected and kept at 400 µmol·mol-1 during the measurement period. After 30 min of dark adaptation, the minimal fluorescence yield (F0) was measured using a low light level (0.005 µmol·m-2·s-1). The maximal fluorescence yield (Fm) was measured using a saturating pulse (8,000 µmol·m-2·s-1) for 1 s on dark-adapted leaves. The leaves were then continuously illuminated for 30 min with actinic light (250 µmol·m-2·s-1) with a red-to-blue light ratio of 9:4 to record the steady-state yield of fluorescence (Fs). The steady-state maximal fluorescence yield (Fm’) was measured using a saturating pulse (8,000 µmol·m-2·s-1) for 1 s on the light-adapted leaves. When the actinic light was turned off, the minimal fluorescence yield (F0’) in the light-adapted state was determined after 5 s of far-red light (25 µmol·m-2·s-1). The difference between the measured values of Fm and F0 was determined as the variable fluorescence (Fv). The chlorophyll fluorescence parameters were calculated using the following formulas:
where Fv/Fm is the maximum quantum efficiency of photosystem II (PSII) and ϕPSII is the actual photochemical efficiency of PSII. The electron transport rate (ETR) is the product of ϕPSII, PPFD, the average light absorption ratio by leaf, and the average PSII to PSI reaction center ratio. NPQ and qP are the non-photochemical and photochemical quenchings, respectively, and qL is the estimation of the fraction of open PSII centers.
Growth Characteristics
Six paprika transplants in each treatment were randomly selected 14 and 28 days after the cooling treatments (DACT). At 14 DACT, the shoot length, stem diameter, number of internodes and leaves, fresh and dry weights of the leaves, stems, and roots, leaf area, and SPAD value were measured. At 28 DACT, the measurement parameters were the same, except that the shoot length and number of internodes were measured for both before and after branching parts of the plants. Stem diameters were measured at the thickest part of each stem using a digital caliper (Mitutoyo, Japan). The number of internodes was counted from the cotyledon to the shoot tip. Internodes were counted if they were longer than 5 mm. The total leaf area of each plant was measured by a leaf area meter (LI-3100, LI-COR, Lincoln, NE, US). Dry weights were measured after drying at 60°C for a week. The average relative chlorophyll content (SPAD value) of four leaves from the 3rd to 6th and 5th to 8th true leaves at 14 and 28 DACT, respectively, was measured using a chlorophyll meter (SPAD 502, Konica Minolta, Sakai, Japan).
Data Collection and Statistical Analysis
The experiment adopted a completely randomized block design with three replications. Statistical Analysis System (SAS) for Windows version 9.4 (SAS Institute Inc., Cary, NC, USA) software was used to perform ANOVA and Tukey’s honestly significant difference tests, with significant differences among treatments evaluated at p < 0.05.
Results
Medium Temperature Changes and Root Electrolyte Leakage
Medium temperature measurements were collected for 20 days and averaged for each treatment. For all treatments, growing media temperatures were affected by a change in the air temperature in the growth chambers (Fig. 3). During the BHP, medium temperatures increased slightly from 23.2 to 25.3°C at both the center and side points in all treatments. During the HP, as the air temperature increased, the cooling systems decreased the medium temperatures in different patterns. In the NSC treatment, the medium temperature increased to 33.0°C and 33.3°C at the center and side points, respectively, similar to that in the No-cooling treatment, which increased to 33.3°C at both points. During irrigation with the chilled nutrient solution, the medium temperature sharply dropped to 25.9°C at the center point and the temperature at the side point decreased to 28.1°C (Suppl. Fig. 1). These temperatures were approximately 4°C and 1°C lower than those in the No-cooling treatment at the center and side points, respectively. In the WCC treatment, medium temperatures were maintained in the 23.3–25.4°C and 22.7–25.1°C ranges, approximately 6–8°C and 8–8.3°C lower than those in the No-cooling treatment at the center and side points, respectively. At 28 DACT, EL in the roots was not significantly different, but its value was greatest in the No-cooling treatment, followed by the WCC and NSC treatments (Fig. 4).
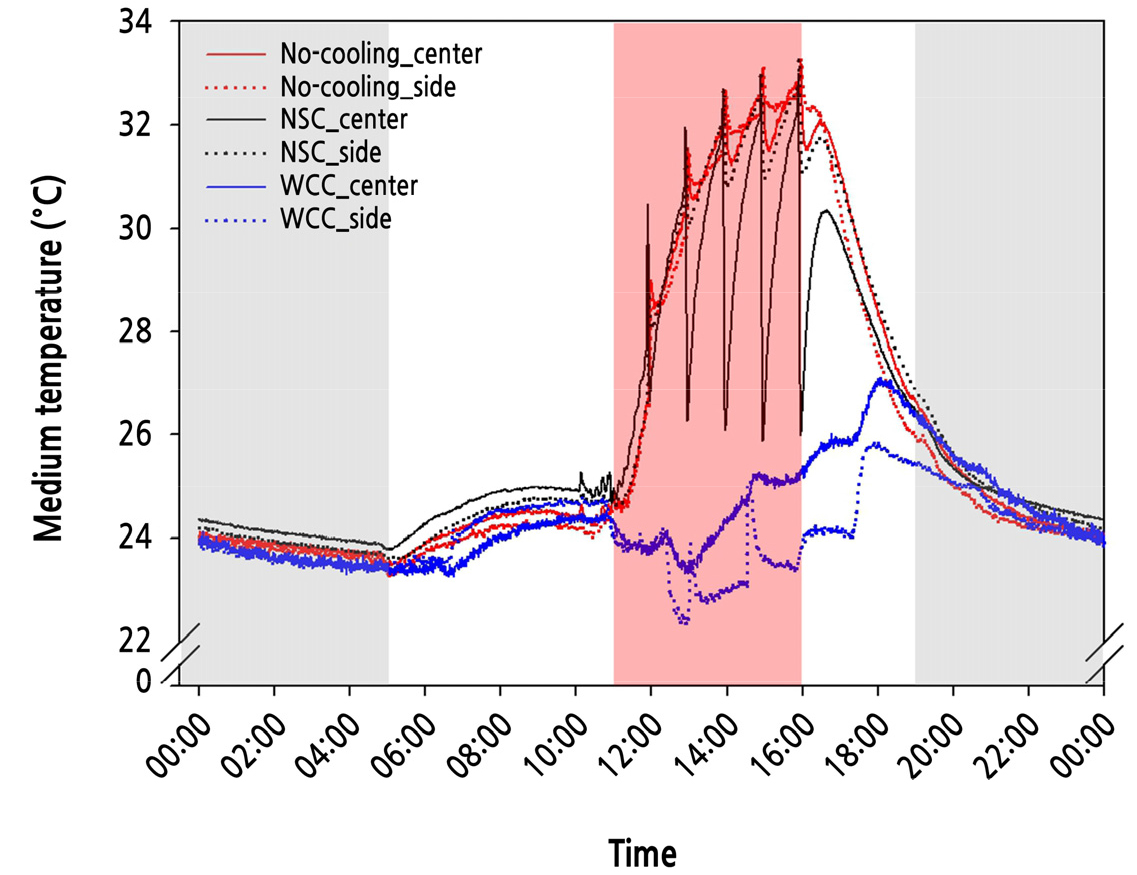
Fig. 3.
The twenty days average temperatures of growing media at center points (solid line) and side points (dotted line) in each treatment: No-cooling (red), NSC (black), and WCC (blue). Gray areas indicate dark periods, and a red area indicates a heating period during a photoperiod represented by the white area from 05:00 to 19:00.
Photosynthetic Traits of the Paprika Transplants
At 13 DACT, An tended to increase as PPFD increased at all chamber temperatures. When the chamber air temperature was maintained at 27°C, the An ranged from –3.4 to –1.9 µmol·m-2·s-1 and from 18.1 to 20.8 µmol·m-2·s-1 at PPFD 0 and 2,000 µmol·m-2·s-1, respectively. An in the cooling treatments was greater than that in the No-cooling treatment, and An in the WCC was 0.89 µmol·m-2·s-1, showing the highest value, while that of the No-cooling treatment was –0.1 µmol·m-2 ·s-1 at a PPFD of 50 µmol·m-2·s-1 (Fig. 5A). Gs and E of the No-cooling treatment tended to decrease sharply in PPFDs from 0 to 100 µmol·m-2·s-1, then gradually decreasing at values above 100 µmol·m-2·s-1. Nevertheless, those in the cooling treatments tended to increase progressively, with no significant differences between NSC and WCC (Fig. 5B and 5C). When maintained at 40°C, An ranged from –5.9 to –4.8 µmol·m-2·s-1 and from 18.6 to 23.2 µmol·m-2·s-1 at PPFDs of 0 and 2,000 µmol·m-2·s-1, which were lower and higher values, respectively, than those measured at an air temperature of 27°C. The An values at PPFDs of 1,000, 1,500, and 2,000 µmol·m-2·s-1 were lowest in the No-cooling treatment, while they were all highest in the NSC treatment (Fig. 5D). Gs and E tended to decrease in all treatments from 0 to 100 µmol·m-2 ·s-1 in PPFD, with NSC showing the greatest decrease, with a subsequent increasing trend. For Gs, the increase was more significant in the cooling treatments than in the No-cooling treatment, and no significant differences were found between the cooling treatments at values above PPFD 500 µmol·m-2·s-1. In addition, E was greatest in NSC and lowest in WCC at 250 µmol·m-2·s-1 PPFD and higher (Fig. 5E and 5F).
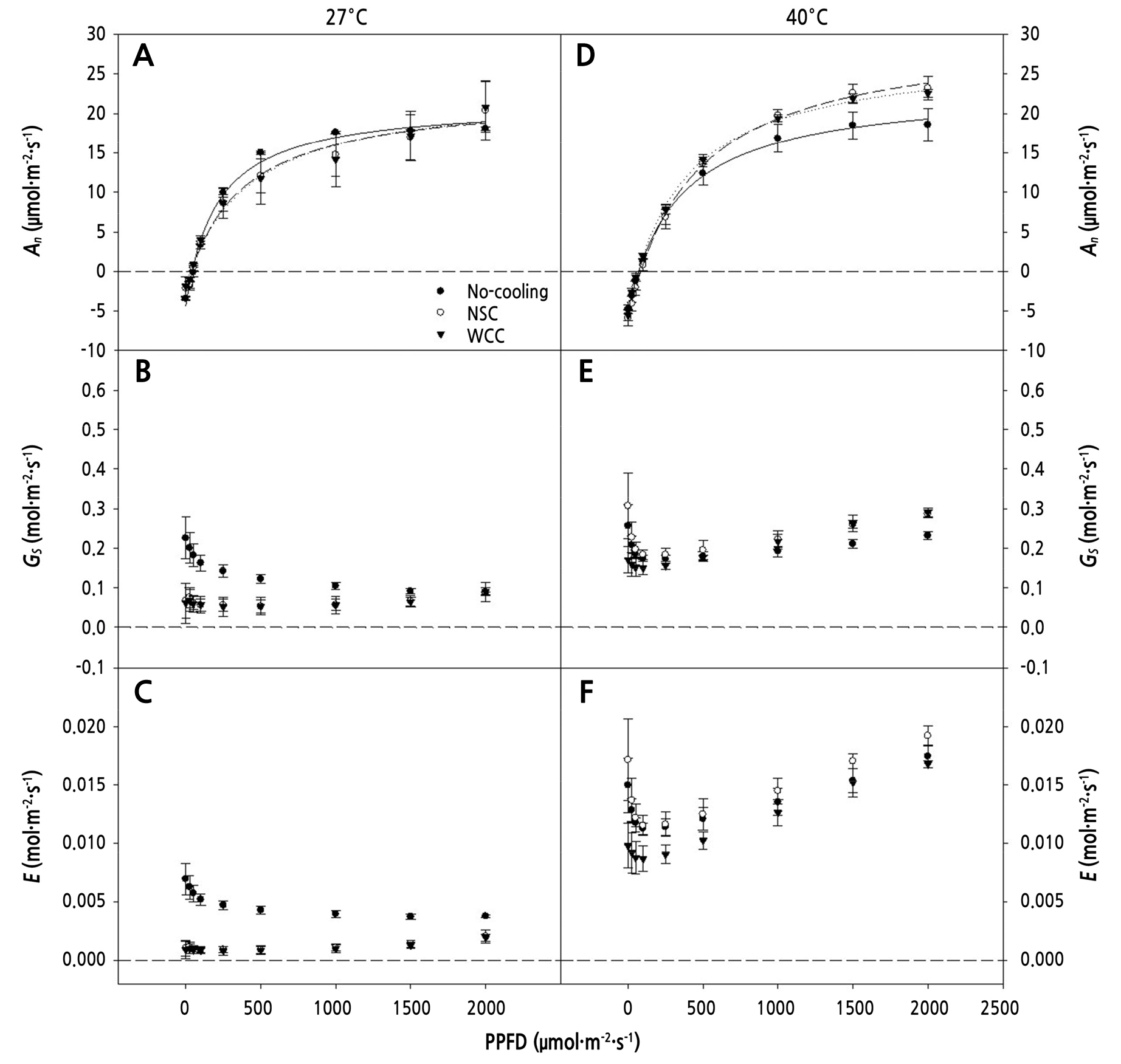
Fig. 5.
CO2 assimilation (An), stomatal conductance (Gs), and transpiration rate (E) at PPFDs ranging from 0 to 2,000 µmol· m-2·s-1 as measured on the fully expanded 4th to 5th leaves from the cotyledon at air temperatures of 27°C (A, B, and C) and 40°C (D, E, and F), respectively, 13 days after cooling treatment. The vertical error bars represent the standard deviations (n = 3).
At 27 DACT, the An values ranged from –2.1 to –1.4 µmol·m-2·s-1 and from 16.5 to 19.1 µmol·m-2·s-1 at PPFD 0 and 2,000 µmol·m-2·s-1, respectively, when the chamber air temperature was maintained at 27°C (Fig. 6A). Gs and E showed no significant differences in any treatment for any PPFD level (Fig. 6B and 6C). When maintained at 40°C, the An values ranged from –3.7 to –2.7 µmol·m-2·s-1 and from 21.2 to 28.0 µmol·m-2·s-1 at PPFD 0 and 2,000 µmol·m-2·s-1, respectively (Fig. 6D). The compensation point of the light response curve (Suppl. Table 1) was greater in the cooling treatments (PPFDs of 53.01 µmol·m-2·s-1 and 55.45 µmol·m-2·s-1 for NSC and WCC, respectively) than in the No-cooling treatment (PPFD of 45.69 µmol·m-2·s-1). As PPFD increased, An in the cooling treatments was greater than that in the No-cooling treatment, which had a trend similar to that at 13 DACT under the same air temperature (Fig. 6D). Similar to the 27°C conditions, Gs and E showed no significant differences among the treatments (Fig. 6E and 6F).
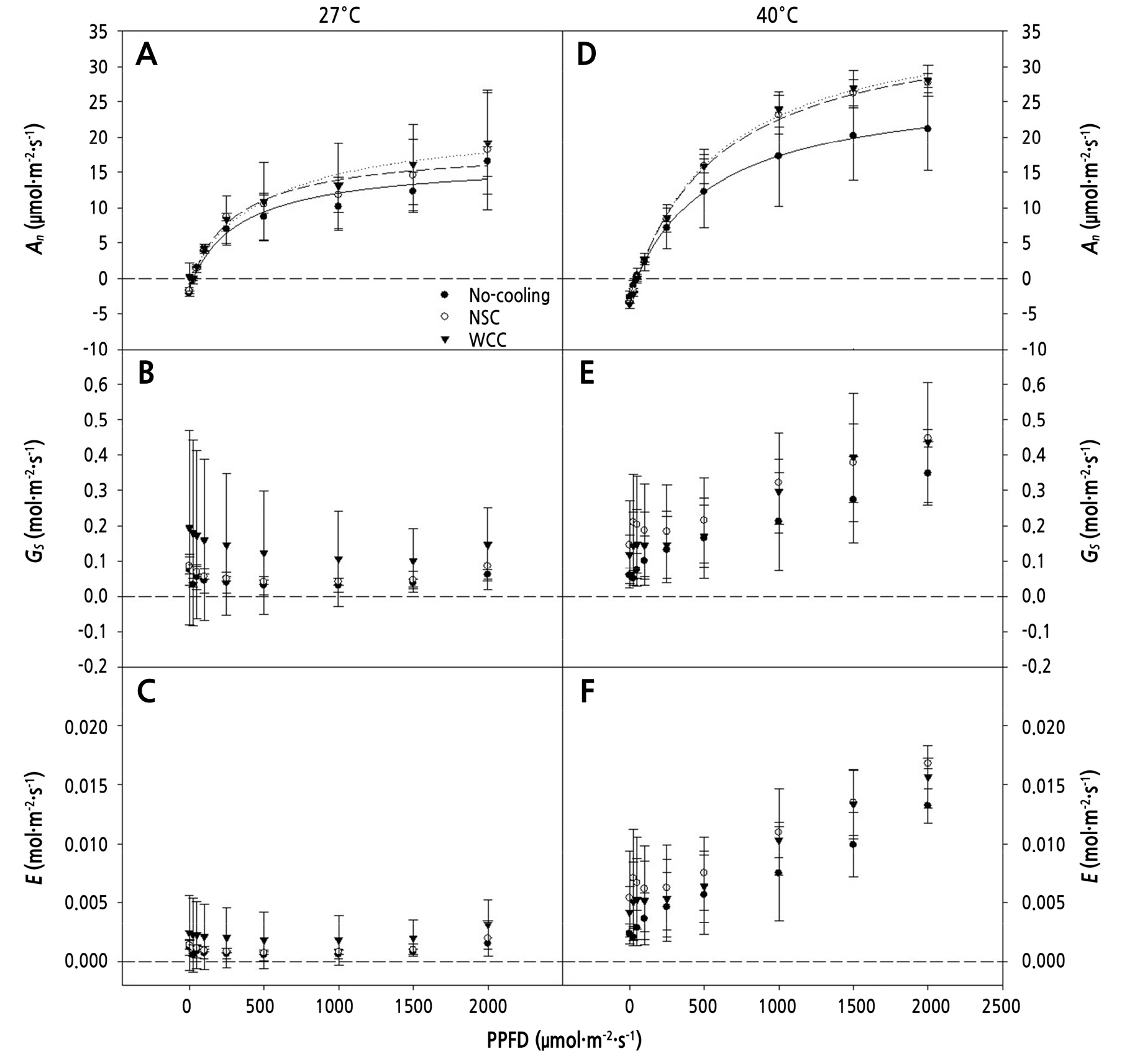
Fig. 6.
CO2 assimilation (An), stomatal conductance (Gs), and transpiration rate (E) at PPFDs ranging from 0 to 2,000 µmol ·m-2·s-1 as measured on the fully expanded 4th to 5th leaves from the cotyledon at air temperatures of 27°C (A, B, and C) and 40°C (D, E, and F), respectively, 27 days after cooling treatment. The vertical error bars represent the standard deviations (n = 3).
Chlorophyll Fluorescence of the Paprika Transplants
The maximal quantum efficiency (Fv/Fm) was lower in the No-cooling treatment than in the cooling treatments, while that of WCC was highest (0.82) at 14 DACT (Fig. 7A). The quantum efficiency of PSII (ϕPSII) was highest (0.64) in the NSC treatment, followed by the No-cooling and WCC treatments (Fig. 7B). The Fv/Fm and ϕPSII showed no significant differences in any treatment at 28 DACT (Fig. 7C and 7D). The electron transport rate (ETR) was highest (66.83) in the NSC treatment, followed by the No-cooling and WCC treatments at 14 DACT (Fig. 8A). The NPQ was greatest in the WCC treatment, followed by the NSC and No-cooling treatments (Fig. 8B). The ETR showed no significant differences (Fig. 8C) and the NPQ was greater in the cooling treatments than in the No-cooling treatment at 28 DACT (Fig. 8D). Photochemical quenching (qP) was greater in the No-cooling and NSC treatments than in the WCC treatment (Fig. 9A) at 14 DACT. The fraction of open PSII centers (qL) was greater in the NSC and No-cooling treatments than in the WCC treatment (Fig. 9B). The qP and qL outcomes showed no significant differences in any treatment (Fig. 9C and 9D).
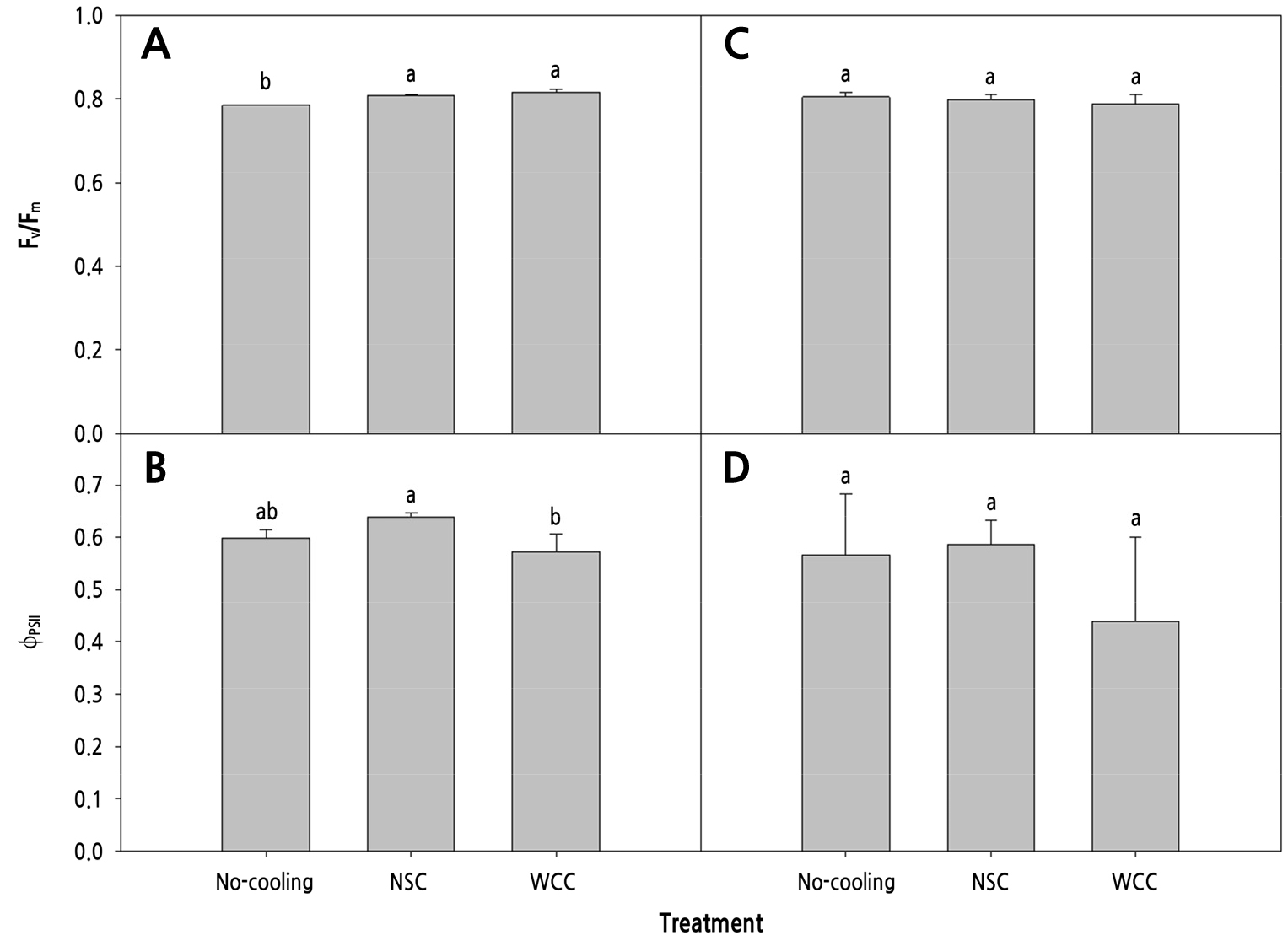
Fig. 7.
The maximum quantum efficiencies of PSII (Fv/Fm) and quantum efficiencies of PSII (ΦPSII) of the 4th to 5th and 8th to 10th paprika transplant leaves treated with different cooling systems during the heating period 14 (A and B) and 28 (C and D) days after cooling treatments, respectively. The data shown are the means (n=12) ± standard deviations. Different letters are considered significant at p < 0.05 by Tukey’s honestly significant difference test.
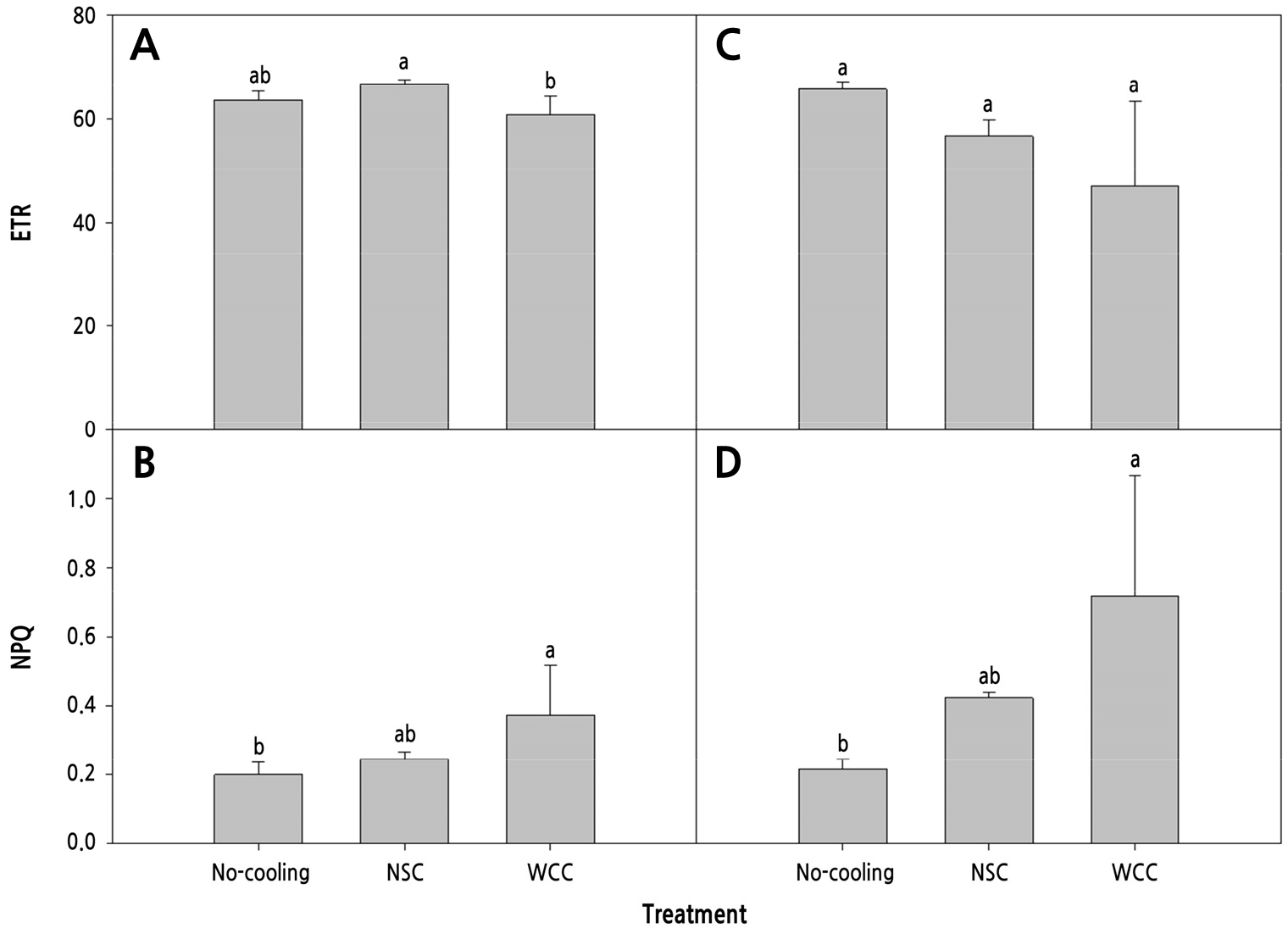
Fig. 8.
Electron transport rate (ETR) and non-photochemical quenching (NPQ) of the 4th to 5th and 8th to 10th paprika transplant leaves treated with different cooling systems during the heating period 14 (A and B) and 28 (C and D) days after cooling treatments, respectively. The data shown are the means (n=12) ± standard deviations. Different letters are considered significant at p < 0.05 by Tukey’s honestly significant difference test.
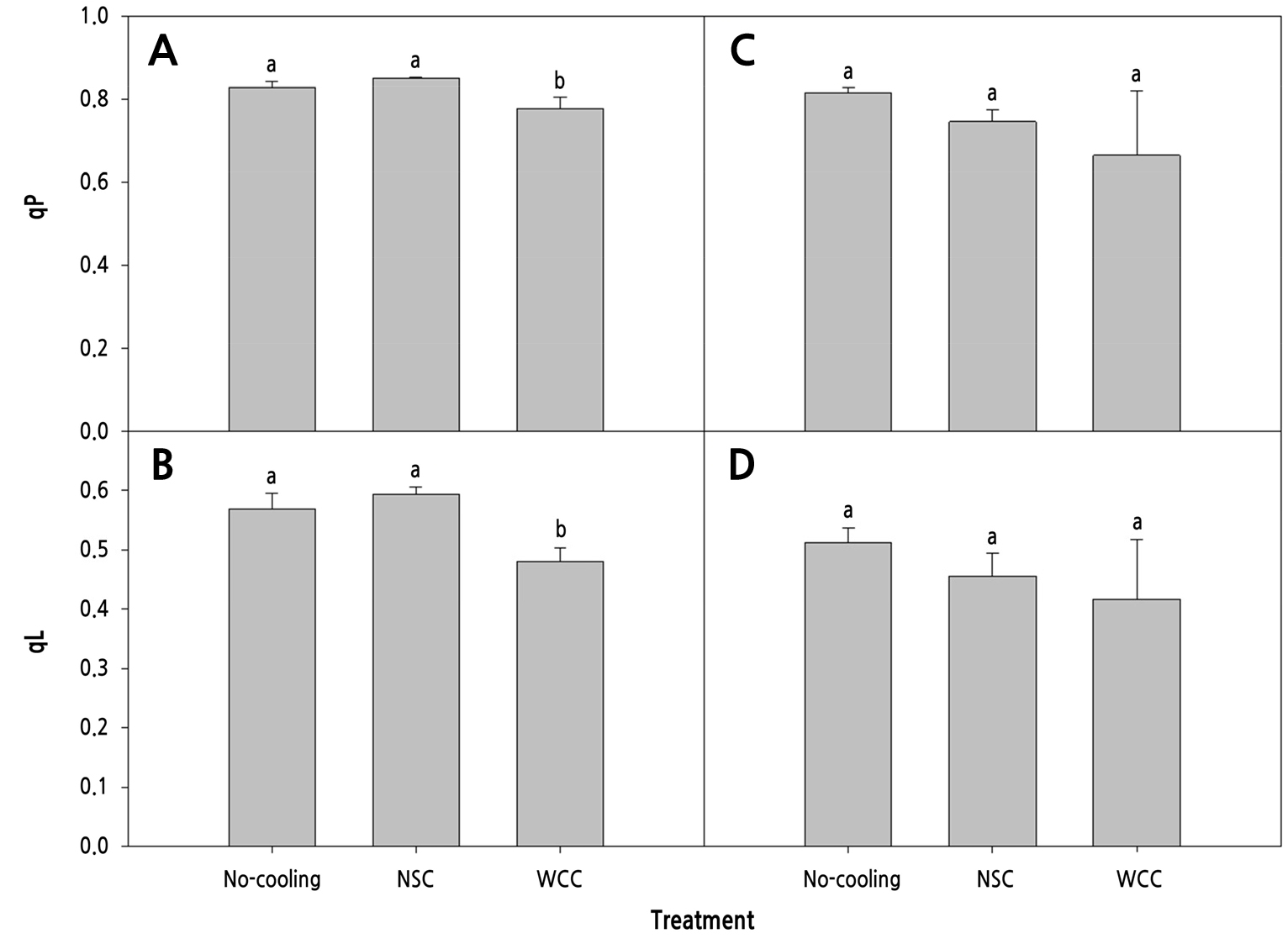
Fig. 9.
Photochemical quenching (qP) and the fraction of open PSII centers (qL) of the 4th to 5th and 8th to 10th paprika transplant leaves treated with different cooling systems during the heating period at 14 (A and B) and 28 (C and D) days after cooling treatments, respectively. The data shown are the means (n=12) ± standard deviations. Different letters are considered significant at p < 0.05 by Tukey’s honestly significant difference test.
Growth of the Paprika Transplants
At both 14 and 28 DACT, the plants in the No-cooling treatment showed more wilting than those in cooling treatment, with leaves becoming shriveled and dropping to a more vertical position (Fig. 10). The stem diameter was greatest in the NSC treatment, followed by the WCC and No-cooling treatments at 14 DACT (Table 1). At 28 DACT, the leaf area was largest in the No-cooling treatment, followed by the WCC and NSC treatments. The number of internodes after branching was greater in the cooling treatments than in the No-cooling treatment. In addition, the SPAD value was greatest in the WCC treatment compared to those of the other treatments (Table 2).
Table 1.
Shoot growth of paprika transplants 14 days after cooling treatment
Treatment | Shoot length before branching (cm) | Stem diameter (mm) | No. of internodes | No. of leaves | SPAD value |
Leaf area (cm2) |
No-cooling | 15.4 az | 6.2 b | 9.2 a | 12.0 a | 48.3 a | 672.4 a |
NSC | 16.2 a | 6.9 a | 10.0 a | 13.8 a | 50.5 a | 756.1 a |
WCC | 15.0 a | 6.7 ab | 9.7 a | 12.7 a | 50.3 a | 633.5 a |
Table 2.
Shoot growth of paprika transplants 28 days after cooling treatment
Treatment |
Shoot length (cm) |
Stem diameter (mm) | No. of internodes | No. of leaves | SPAD value |
Leaf area (cm2) | ||
Before branching |
After branching |
Before branching |
After branching | |||||
No-cooling | 17.1 az | 11.5 a | 9.3 a | 7.3 a | 4.0 b | 35.2 a | 47.6 b | 2392 a |
NSC | 17.6 a | 10.8 a | 9.7 a | 7.5 a | 4.5 ab | 35.7 a | 47.8 b | 2082 b |
WCC | 17.5 a | 10.6 a | 9.5 a | 7.5 a | 4.7 a | 36.3 a | 50.8 a | 2300 ab |
The stem dry weight was greatest in the NSC treatment, while root dry weight was greater in the cooling treatments than in the No-cooling treatment at 14 DACT. The shoot-to-root ratio was higher in the No-cooling and NSC treatment than in the WCC treatment. The root dry weight was greatest in NSC treatment, followed by the WCC and No-cooling treatments, and the shoot-to-root ratio was lowest in NSC, followed by the WCC and No-cooling treatments at 28 DACT (Table 3). The dry weight distributions in leaves and stems were greater in the No-cooling and NSC treatments than in the WCC treatment; however, that for the roots was greatest in the WCC treatment. The dry weight distribution in the leaves was greatest in the No-cooling treatment, followed by the WCC and NSC treatments, however, that in the root was greatest in the NSC treatment, followed by the WCC and No-cooling treatments (Table 4).
Table 3.
The leaf, stem, and root dry weights and the shoot-to-root ratios of paprika transplants 14 and 28 days after cooling treatment
Treatment | Dry weights (g/plant) | Shoot-to-root ratio | |||
Leaf | Stem | Root | Total | ||
14 days | |||||
No-cooling | 1.7 az | 0.6 b | 1.6 b | 3.9 b | 1.4 a |
NSC | 2.1 a | 0.8 a | 2.1 a | 5.0 a | 1.4 a |
WCC | 1.7 a | 0.6 b | 2.2 a | 4.6 ab | 1.0 b |
28 days | |||||
No-cooling | 6.7 a | 4.2 a | 4.2 b | 15.1 a | 2.6 a |
NSC | 5.9 a | 3.9 a | 5.0 a | 16.1 a | 2.0 b |
WCC | 6.5 a | 4.0 a | 4.6 ab | 14.8 a | 2.3 ab |
Table 4.
The leaf, stem, and root dry weight distributions and specific leaf areas (SLA) of paprika transplants 14 and 28 days after cooling treatment
Treatment | Dry weight distribution (% of whole plant dry weight) |
SLA (mg/cm2) | ||
Leaf | Stem | Root | ||
14 days | ||||
No-cooling | 43.0 az | 15.6 a | 41.5 b | 2.5 a |
NSC | 41.7 a | 16.2 a | 42.1 b | 2.7 a |
WCC | 37.4 b | 13.5 b | 49.1 a | 2.7 a |
28 days | ||||
No-cooling | 44.5 a | 27.9 a | 27.6 b | 2.8 a |
NSC | 39.9 b | 26.2 a | 33.9 a | 2.9 a |
WCC | 43.0 ab | 26.6 a | 30.5 ab | 2.8 a |
Discussion
Temperature data of the medium confirmed that during cultivation with small volumes of the medium, similar to most commercial hydroponic systems currently in use, a high air temperature (39°C) can easily increase the root-zone temperature up to 33°C (Fig. 3). The EL of the roots in the No-cooling treatment reached more than 80% after 24-h of incubation, showing that the roots of paprika plants under such temperature condition had suffered heat stress (Fig. 4). This may lead to a decrease in the root dry weight with morphological changes in the shoots according to the decreases in the numbers of internodes after branching as plants are exposed to high air temperatures for more extended periods (Fig. 10; Tables 2, 3, and 4). The root growth and shoot morphological traits would be detrimental to light distribution and biomass production (Sarlikioti et al., 2011), negatively affecting the vegetative growth in the roots and shoots after transplanting. Plants are damaged when the RZT exceeds 40°C for several hours (Ingram et al., 1986; Ruter and Ingram, 1990; Mathers, 2003). As a function of the thermostability of the cell membrane, EL was analyzed to measure the degree of organ sensitivity to heat stress (Bajji et al., 2002). Paprika plants exposed to more extended high air temperature periods had lower root activity due to increased medium temperatures (Choi et al., 2014). As the root zone was exposed to high air temperatures without RZCs, root viability was reduced, as indicated by the higher EL level (Fig. 4).
Besides the effects on roots, a high air temperature also induces leaf moisture loss. This can combine with the reduced water absorption due to heat stress on the roots, causing the plant to lose water and to wilt, with a decrease in the photosynthetic capability of the leaf. At 13 DACT, Gs and E were greater in the No-cooling than in the cooling treatments for all PPFDs (Fig. 5B and 5C), but the An values were not significantly different (Fig. 5A). In addition, Fv/Fm and ϕPSII were lowest in the No-cooling treatment (Fig. 7A and 7B). When the chamber temperature was set to 40°C during HP, leaf photosynthesis was greater in the cooling treatments, and the differences between the No-cooling and cooling treatments gradually increased as the PPFD was increased from 250 and 100 µmol·m-2·s-1 at 14 and 28 DACT, respectively (Fig. 5A and 5D). This increased leaf photosynthesis may stem from the greater root development by RZCs during HP (Tables 3 and 4). When the paprika plants were grown at an air temperature of 33°C, An and Gs were greater, and the vegetative growth was improved compared to plants grown at 25°C (Erickson and Markhart, 2001). As the PPFD increased, Gs and E tended to increase, and the mean values were higher in the cooling treatments than in the No-cooling treatment at an air temperature of 40°C (Fig. 6E and 6F). As the medium temperature decreased to 24.7°C under a high air temperature, which was 3.5°C lower than that of the control, An, E, and Gs all increased (Choi et al., 2013). Leaf temperatures tended to be greater than the ambient air temperature when the RZT was high, suggesting that the plants lacked moisture or were water-stressed (Li et al., 2014). Conversely, if the leaf temperature is lower than the ambient air temperature, E would be greater, and the plant would show high energy consumption. In other words, the Gs and E values of paprika leaves increased at an air temperature of 40°C via the RZC treatments, suggesting that the photosynthetic traits of the leaves improved.
Two RZC systems were applied in this study. In the NSC treatment, the nutrient solution was chilled to 15.9 ± 0.8°C in a reservoir, and its temperature range was 15.1–16.7°C. When irrigation started and the chilled nutrient solution reached the centers in the coir, the medium temperature decreased sharply from more than 30°C to approximately 26°C. Nonetheless, after the termination of irrigation, it returned to the initial medium temperature at a relatively slow speed (Fig. 3). During irrigation with the chilled nutrient solution, the heat may have been conducted to the water attached to the coir substrate, pouring toward the ground as gravitational water through the growing medium. However, the amount of nutrient solution applied to the ambient plant roots stretched through the coir substrate may not have been enough to transfer energy to cool down for an extended time. In this study, the nutrient solution was irrigated five times in total during the HP, once an hour (100 mL), to prevent excessive moisture conditions in the coir substrate with draining to another tank. The idea behind this intermittent cooling method stemmed from the fact that plants were observed to be able to tolerate heat stress for a certain duration (Marias et al., 2017); therefore, periodical short-term cooling could be enough to help the plants recover from the heat stress. For cooling, one or two irrigation periods with more nutrient solution would be advantageous to decrease the medium temperatures. When the NSC system was applied to pepper plants using hydroponics, their growth was accelerated in the chilled nutrient solution (20°C) treatment as compared to the non-chilled case (30°C) (Dodd et al., 2000). The nutrient solution temperature is directly related to the oxygen consumed by the plant (Trejo-Téllez and Gómez-Merino, 2012). When the nutrient solution temperature exceeded 22°C, an increase in the gas diffusion rate resulted in insufficient oxygen demand of the roots, leading to increased vegetative growth and reduced reproductive growth in tomatoes (Graves, 1983). Despite the drastic changes in the medium temperature over the short term in the NSC treatment during HP (Suppl. Fig. 1), the EL of roots in the NSC was highest (Fig. 4) and the medium temperature returned to the ambient air temperature (24–25°C) for more extended periods, including DP and BHP compared to HP. In addition, short-term cooling (for one or two minutes) to 26°C by NSC resulted in greater root growth and biomass levels than in the No-cooling treatment (Table 3). The chilled nutrient solution was applied directly to the roots, which may have provided more oxygen to the roots and promoted root respiration to provide energy for root growth.
Compared to the direct method of cooling the root zone via NSC, the WCC system is an indirect way to reduce the substrate temperature without affecting the substrate water content. In the WCC treatment, the water was chilled to 15.9 ± 0.8°C and circulated for 45 L·min-1 by a submerged electric pump. When the chilled water entered the pump, its inlet temperature range was 15.0–16.8°C, increasing to 16.2–18.2°C after circulation. The temperature difference was in the range of 0.9–1.8°C, indicating that the chiller may consume more energy (2,194 kcal·d-1) than that in the nutrient solution supplied daily per plant (4.55 kcal·d-1) in the NSC treatment when the energy was consumed in relation to the coolant, affecting the medium temperature changes. When the chilled water was circulated, the temperature of the coir substrate decreased to 23°C in the HP (Fig. 3). The silicone pipe was tightly coiled inside of the pot to minimize the temperature differences in the coir substrate, and a slight temperature difference (< 1°C) between the center and side points was found in the WCC treatment (Fig. 3). It is therefore necessary to design an effective cooling system that considers the energy used to lower the substrate and plant temperatures. With regard to management, the parts that conduct heat should be insulated to maintain the growing media and plant temperatures and to save energy. WCC via an X-L pipe was shown to decrease the temperature of a perlite substrate, and the temperature difference was correlated with the depth (Lee et al., 2002). When the coir substrate was cooled via an X-L pipe at 20°C water, 24-h of cooling was suggested, rather than night-time cooling, to overcome the late paprika fruit set caused by the high greenhouse air temperature (Choi et al., 2014). A WCC system using a plastic pipe with low thermal conductivity (0.3 kcal·m-1·h-1·k-1) improved the cooling efficiency compared to a stainless steel pipe with high thermal conductivity (22.0 kcal·m-1·h-1·k-1) in terms of heat loss. The root dry weight was 1.5-fold greater, and the RZT was reduced to 3.6°C in the WCC treatments compared to that in the No-cooling treatment of paprika transplants (Kwack et al., 2014). A silicone pipe with a lower thermal conductivity (1.7 kcal·m-1·h-1·k-1) than a plastic pipe would reduce the heat loss when the water is circulated during the WCC treatment. Energy could be used more efficiently if the WCC system minimizes the heat loss while the water circulates through the pipe and focuses heat transfer only on the target, such as growing media.
In conclusion, the two RZC methods (WCC and NSC) assessed here proved effective in lowering medium temperatures, resulting in greater root growth and leaf photosynthesis in paprika plants. Furthermore, the NSC approach was simpler to apply than the WCC method; it did not require the construction of a complicated circulation pipe system, constant cooling, or energy input yet could more effectively improve root growth during the summer.
Supplementary Material
Supplementary materials are available at Horticultural Science and Technology website (https://www.hst-j.org).
- HORT_20220061_Table_1s.pdf
Light response curves and their equations and coefficient square values by treatments at 27°C and 40°C air temperatures in the chamber 13 and 27 days after cooling treatment
- HORT_20220061_Figure_1s.pdf
The 20-day average temperatures of growing media at dripper points (solid line) and side points (dotted line) in each treatment: No-cooling (red), NSC (black), and WCC (blue) from 14:50 to 16:10 which shorten the time scale from Fig. 3.