Introduction
Materials and Methods
Preparation of Plant Materials and CNM Treatments
CNM Treatments
Relative Water Content and Relative Electrolyte Leakage
Detection of Physiological Indices
Hydroxyl Radical Prevention and DPPH Free Radical Scavenging Assay
Statistical Analysis
Results
Morphological Characteristics of Carnation Cut Flowers under CNM Treatments
Detection of the Relative Water Content and Cell Membrane Stability
Determination of ROS, the Activity of the Antioxidant System, and Lipid Peroxidation
Free Radical Scavenging Ability Test
Discussion
Conclusions
Introduction
Nanotechnology has been widely utilized in diverse fields, such as electronics, medicine, communications, agriculture, personal care, and wastewater treatment. Engineered nanomaterials (NMs) include carbon-based (carbon nanotubes, graphene, and fullerenes) and metal-based NMs (Zuverza-Mena et al., 2017). The minute size of these NMs make their chemical actions entirely distinct from the parent material (Brunner et al., 2006). NMs not only possess high surface area, high strength, and structural stability but also exhibit outstanding thermal and electrical conductivities (Geim and Novoselov, 2007; Liu et al., 2017). Recently, the potential role of nanotechnology in improving crop productivity has been recognized (Rosa et al., 2017).
Some reports have confirmed that NMs can induce positive or negative effects on plants. NMs can influence key biological processes of plants such as photosynthesis, seed germination, root growth, and resistance to oxidative stress (Yang et al., 2006; Heinen et al., 2009; Khodakovskaya et al., 2009, 2011, 2012; Smirnova et al., 2012). The positive effect of NMs on promoting seed germination can be explained by its role in regulating aquaporins (water channels) (Heinen et al., 2009; Khan et al., 2017). Khodakovskaya et al. (2012) indicated that the expression of the tobacco (Nicotiana tabacum) aquaporin (NtPIP1) gene, as well as production of NtPIP1 protein, significantly increased in cells exposed to carbon nanotubes compared to control cells. The enhanced accumulation of water channel proteins by carbon nanotubes has also been validated in soybean (Glycine max), corn (Zea mays), and barley (Hordeum vulgare) (Lahiani et al., 2013). By contrast, some studies have shown toxicological effects of NMs on plants, which could lead to the production of reactive oxygen species (ROS), causing an imbalance of the cell redox status and cell damage (Wang et al., 2014; Chichiricco and Poma, 2015). Thus, the role of NMs in improving plant growth and development and stress tolerance is controversial. Dietz and Herth (2011) elucidated that the toxicity of NMs to plants depends on their properties, size, concentration, and subcellular localization. Khan et al. (2017) reported that the toxicity of most NMs occurred at higher concentrations, and lower concentrations showed beneficial or no effect on plant biological processes.
Carbon-based NMs (CNMs) have gained increasing attention due to their favorable biocompatibility and special chemical, physical, and electrical properties (Gao et al., 2017). Graphene, a one-atom-thick, two-dimensional sheet, exhibits the highest mechanical strength, extraordinary electron transfer capacity, and unprecedented pliability and impermeability (Lan et al., 2017). Furthermore, graphene can be rolled to form nanotubes or wrapped to form fullerenes (С60 and С70) and graphene quantum dots (GQDs) (Ghuge et al., 2017). CNMs can also be used to influence plant growth, development, and stress tolerance, and some CNMs have been widely applied as electrochemical biosensors to detect H2O2, H2S, and NO inside cells depending on their electrocatalytic activities (Liu et al., 2017). For example, carbon nanotubes (CNTs) delayed flowering and affected seed formation in rice (Oryza sativa) (Lin et al., 2009), and affected the expression of stress-related genes and peroxidase (POX) activity in tomato (Solanum lycopersicon) seedlings (Khodakovskaya et al., 2011; Smirnova et al., 2012). Giraldo et al. (2014) found that single-walled carbon nanotubes (SWCNTs) increased photosynthesis by three-fold and significantly elevated the electron transfer rate by reducing chloroplast ROS generation. Fullerene can quench free radicals, thereby having a cellular protective effect, or produce ROS to induce cell damage (Markovic and Trajkovic, 2008). Zuverza-Mena et al. (2017) reported that all CNMs could change the expression level of stimuli-related genes. Some studies exhibited that NMs that increase ROS generation play dual roles in plants including functioning as toxic compounds and signaling molecules.
ROS generation is a common response of plant cells under stresses. ROS include superoxide anion radicals (O2·-), hydrogen peroxide (H2O2), singlet oxygen (1O2), and hydroxyl radicals (OH·), which are highly reactive. Low concentrations of ROS act as signaling molecules to regulate cellular defense systems, and excessive ROS can cause oxidative stress and cell damage (Tatone et al., 2010). Plants have complex antioxidant systems including both enzymatic and nonenzymatic antioxidants to scavenge harmful ROS (Khan et al., 2017). Among the enzymatic antioxidants, superoxide dismutase (SOD) and catalase (CAT) represent the first lines of defense against ROS (Van Breusegem et al., 2001). SOD catalyzes O2·- to form H2O2, and CAT further metabolizes H2O2 into H2O and O2. Additionally, O2·- and H2O2 can be converted to generate OH· by the Haber–Weiss cycle and Fenton reaction (Zhang et al., 2015). Among the nonenzymatic antioxidants, ascorbic acid (AsA) is a powerful ROS scavenger, and glutathione (GSH) is necessary to counteract ROS-induced oxidative stress and maintain the normal reduced state of cells (Meyer, 2008). The AsA–GSH cycle plays a crucial role in the antioxidant system (Foyer and Halliwell, 1976; Gill and Tuteja, 2010).
Carnation (Dianthus caryophyllus L.) is a popular floriculture crop used for cut flowers. Vase life (VL) is an important characteristic of cut flowers. VL is defined as the duration from the flower cut off the plant and put into the vases to the loss of ornamental value due to senescence. A gradual reduction in water uptake caused by the obstruction of xylem vessels is a major factor for reduced VL. Microbial growth; deposition of lignin, suberin, or tannin in the lumen of xylem vessels; and air embolisms in the vascular system also reduce water uptake (Boxriker et al., 2017a). Increasing VL involves slowing the senescence process and avoiding rapid dehydration of the plant tissue (Ebrahimzadeh et al., 2008; Oh and Lee, 2020). The processes of senescence and abscission are accompanied by various stresses, such as decreased antioxidant enzymes activity, accumulation of ethylene and ROS, and membrane damage (Prochazkova and Wilhelmova, 2007; Kim et al., 2020). Cut flowers are ideally suited for the study of plant cell senescence and/or stress acclimation, and are commonly used to test the antioxidative defense status, postharvest stresses, and phytotoxicity of chemicals. In this study, we detected ROS metabolism, the activity of the antioxidant system, and the stress response of carnation cut flowers treated with different CNMs to reveal whether the CNMs have antioxidative or phytotoxicity effects on plant cells.
Materials and Methods
Preparation of Plant Materials and CNM Treatments
Carnation (Dianthus caryophyllus L. ‘Damina’) plants were grown in the greenhouse of flower planting base of Qingpu District, Shanghai, China. The flowers were harvested before blooming in the fall of 2016 and immediately brought to the laboratory.
CNM Treatments
CNMs were purchased from Nanjing XFNANO Materials Tech Company, China. The CNMs included SWCNTs aqueous solution (0.5 g·L-1, particle diameter 1 nm, length 1 µm), C60 aqueous solution (0.5 g·L-1, particle diameter 0.7 nm) and GQDs aqueous solution (0.5 g·L-1, particle diameter 3-5 nm, thickness 1 nm).
Each flower stalk was trimmed to a length of 40 cm and placed into a culture bottle filled with different concentrations (1, 5, and 25 mg·L-1) of SWCNT, GQD, or C60; distilled water served as the control group. Each treatment comprised 5 replicates of 15 cut flowers, and supplemented with the CNM solution every 5 d. The treated cut flowers were placed in a plant incubator with a 14-h photoperiod of 3600 Lux, air temperature (25°C/15°C, day/night), and 60-80% relative humidity. The vase life ended when at least 50% of the flowers per treatment were senesced. The blooming process of carnation cut flowers in this study was divided into 5 chronological stages according to Boxriker et al. (2017b) (Fig. 1). The flowers were photographed and the degree of blooming and wilting (Fig. 1) was recorded every day. Three flowers per treatment were collected weekly, the petals were weighed and used for relative water content (RWC) and relative electrolyte leakage (REL) analyses; other materials were stored at -80°C until use.
Relative Water Content and Relative Electrolyte Leakage
The fresh weight (FW) of carnation petals was detected immediately after sampling. The turgid weight (TW) was measured after placing the petals in distilled water for 24 h. The dry weight (DW) was determined by drying the petals at 60°C for 48 h until a constant weight was obtained. The RWC = [(FW-DW) / (TW-DW)] × 100%.
The petal materials (0.2 g) were thoroughly rinsed with deionized water, and then were placed in 20 mL of deionized water and incubated for 2 h. The initial electrical conductivity (C1) was measured using a conductivity meter (Mettler Toledo, Switzerland). The petal was then boiled for 20 min, and the electrical conductivity was measured again after cooling to room temperature (C2). The REL = C1/C2 × 100%.
Detection of Physiological Indices
Detection of malondialdehyde (MDA), H2O2, AsA, and GSH contents; OH· generation activity; O2·- production activity; and SOD, CAT, and peroxidase (POD) activities was performed using biological assay kits (Nanjing Jiancheng Bioengineering Institute, China) following the manufacturer’s instructions. Each detection assay was performed in triplicate.
Hydroxyl Radical Prevention and DPPH Free Radical Scavenging Assay
The hydroxyl radical (OH·) inhibition effect was detected according to the method in Yang et al. (2019). The 2,2-diphenyl-1-picrylhydrazyl (DPPH) free radical scavenging assay of CNMs was performed according Rikabad et al. (2019).
Statistical Analysis
The significant differences (p < 0.05) and correlation analysis (p < 0.05) were performed using SAS 9.1.3 software. For all quantitative data, all the values were described as the means ± standard errors of the means. One-way ANOVA was used, followed by the LSD multiple range test.
Results
Morphological Characteristics of Carnation Cut Flowers under CNM Treatments
GQD and C60 showed better effects in increasing the VL of carnation cut flowers than the SWCNT and distilled water treatments. The average VL was approximately 23.5 d for the distilled water-treated cut flowers, and most flowers entered the beginning blooming and wilting stages on the 3rd and 13th d, respectively (Fig. 1A and 1C). Additionally, 25 mg·L-1 GQD increased the VL by 10.64% compared with that in the control group. However, 1 and 5 mg·L-1 GQD treatments decreased the VL by nearly 5% (Fig. 2A and Table 1). For the C60-treated group, 1 mg·L-1 C60 showed the best effect on slowing senescence, and 5 and 25 mg·L-1 C60 accelerated this process by approximately 4.26% and 8.51%, respectively (Fig. 2B and Table 1). A high concentration of SWCNT promoted flower wilting with a 27.66% decrease in VL, and the VL showed no significant differences between the low-concentration SWCNT treatment and the control (Fig. 2C).
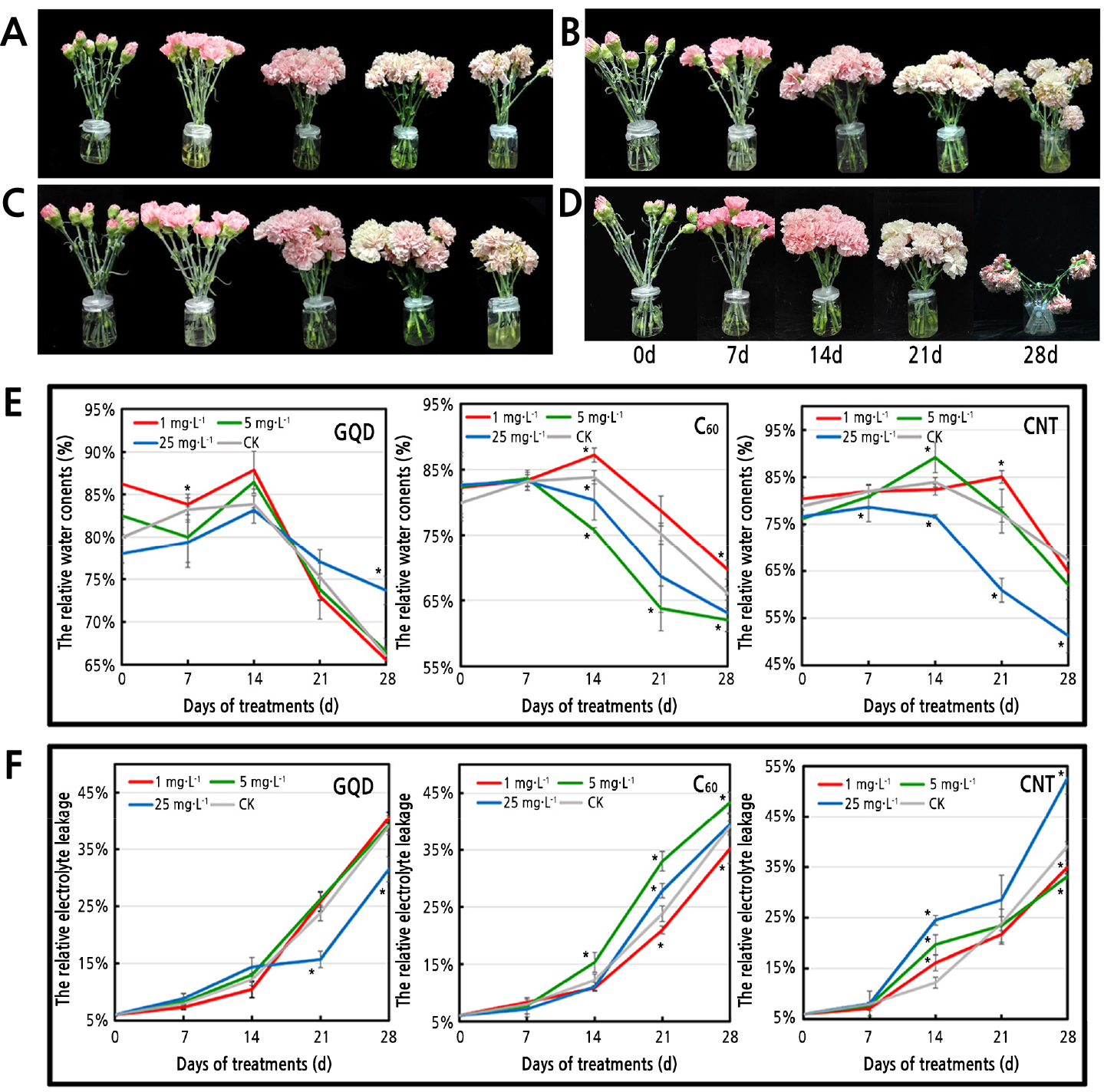
Fig. 2.
Changes in flower developmental stages, relative water content, and relative electrolyte leakage of cut flowers treated with different CNMs. 25 mg·L-1 GQD (A), 1 mg·L-1 C60 (B), 1 mg·L-1 SWCNT (C), distilled water (D), detection of the relative water content of cut flowers (E), and detection of the relative electrolyte leakage of cut flowers (F). CNMs: carbon nanomaterials, CNT: single-walled carbon nanotubes, GQD: graphene quantum dots, C60: fullerene. Each experiment was repeated 3 times, vertical bars represent standard error of the mean (± SE, n=3), and asterisks indicate significant differences between treated and control groups (p < 0.05, least significant difference test).
Table 1.
The Effect of carbon nanomaterials (CNMs) on vase life (VL) of cut carnation flowers
Test group | Treated concentration (mg·L-1) | VL (d) | VL change (%) |
distilled water | - | 23.5 ± 0.25 | 0 |
SWCNT | 1 | 24.5 ± 0.38 | 4.26 |
5 | 23.0 ± 0.63 | -2.12 | |
25 | 17.0 ± 1.44 | -27.66 | |
GQD | 1 | 22.0 ± 0.63 | -6.38 |
5 | 23.0 ± 0.38 | -2.12 | |
25 | 26.0 ± 0.5 | 10.64 | |
C60 | 1 | 25.5 ± 0.25 | 8.51 |
5 | 22.5 ± 0.38 | -4.26 | |
25 | 21.5 ± 0.75 | -8.51 |
Detection of the Relative Water Content and Cell Membrane Stability
The RWC and REL of petals indicated that dehydration and membrane damage of the cells significantly increased after 14 d in each group (Fig. 2). Dehydration and membrane damage of cut flowers obviously decreased in the 25 mg·L-1 GQD treatment compared with the control during the latter 3 stages. The RWC was increased by more than 11%, and the REL was decreased by approximately 22.9% in the GQD-treated group, with no significant differences between the 1 and 5 mg·L-1 GQD treatments and the control (Fig. 2E and 2F). However, 25 mg·L-1 SWCNT markedly accelerated dehydration and membrane damage. In the 1 and 5 mg·L-1 SWCNT treatments, the trends of RWC and REL were similar to those of the control (Fig. 2C). Furthermore, 1 mg·L-1 C60 showed better effects on protecting the cell against dehydration and membrane damage during the whole wilting process, and the REL of petals was decreased by nearly 10% (Fig. 2B and 2F). However, the 5 and 25 mg·L-1 C60 treatments showed the opposite effect. The above results suggest that 25 mg·L-1 GQD and 1 mg·L-1 C60 have positive effects on delaying the senescence of carnation cut flowers.
Determination of ROS, the Activity of the Antioxidant System, and Lipid Peroxidation
Membrane lipid peroxidation detected by MDA showed that the membrane damage of cut flowers continuously increased throughout the experiment, and 1 mg·L-1 C60 and 25 mg·L-1 GQD significantly decreased membrane lipid peroxidation up to 45% in the first 20 d (Fig. 3A). ROS levels also changed obviously in the different treatment groups. The H2O2 content peaked on the 14th d in each treatment group. The H2O2 level of the GQD group was significantly higher than that of the distilled water control, and the H2O2 content of the C60 treatment group was lower than that of the distilled water control (Fig. 3B). O2·- production gradually declined and OH· generation activitycontinuously increased in each treatment group. O2·- production showed a similar trend in the C60 and distilled water groups. However, the O2·- production of GQD-treated petals was significant higher, by approximately 12.5-24.8%, than that of the distilled water control (Fig. 3C). Additionally, the generation of OH· significantly decreased in the CNM treatments compared with that in the distilled water control, and the GQD treatments showed a more obvious effect (Fig. 3D). These results indicated that GQD and C60 affect ROS metabolism during the senescence of carnation cut flowers.
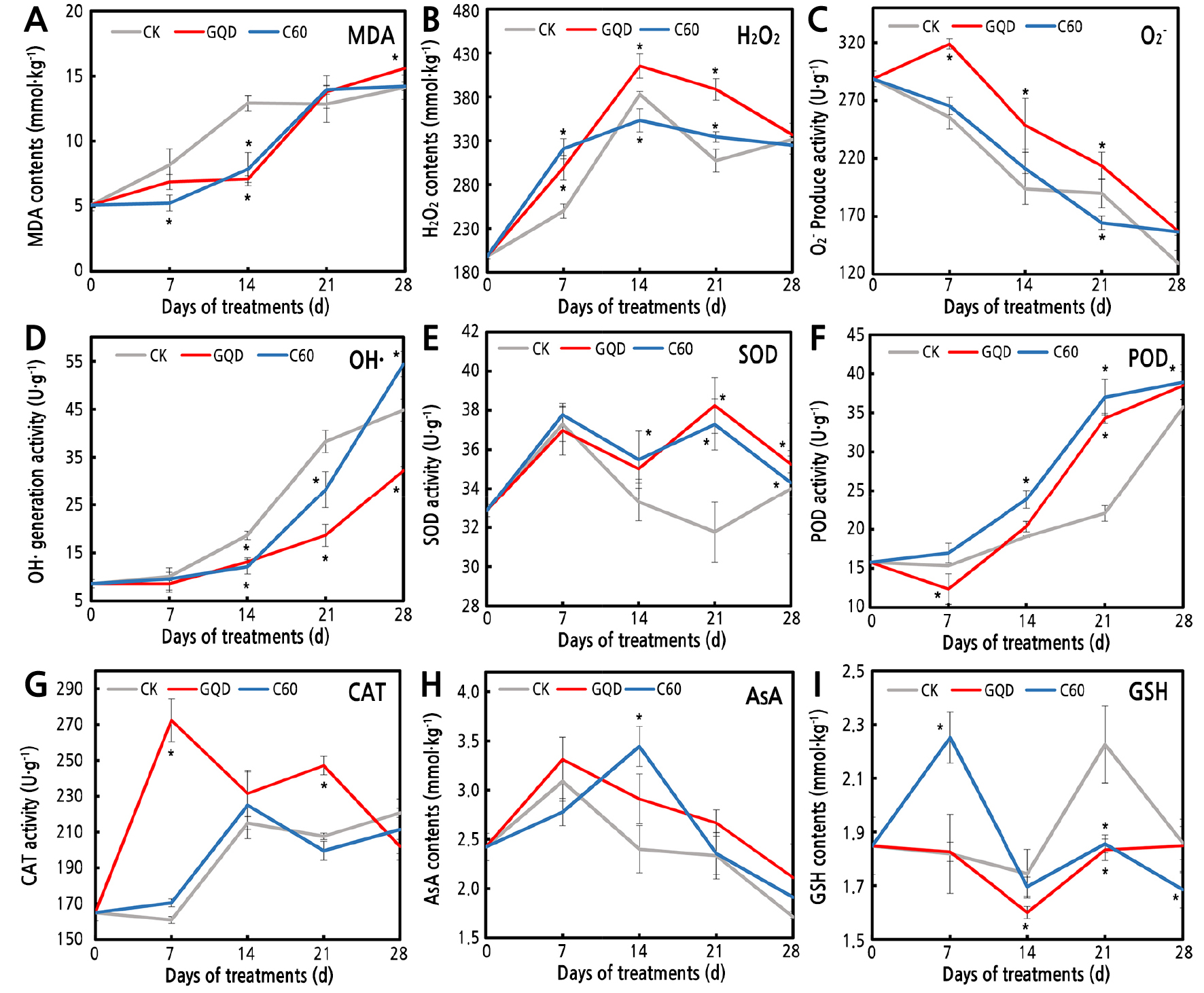
Fig. 3.
Detection of membrane lipid peroxidation, ROS, and activity of the antioxidant system of cut flowers treated with different CNMs. MDA contents (A), H2O2 contents (B), O2·− production (C), OH· generation (D), SOD activity (E), POD activity (F), CAT activity (G), AsA contents (H), and GSH contents (I). Each experiment was repeated 3 times, vertical bars represent standard error of the mean (± SE, n=3), and asterisks indicate significant differences between treated and control groups (p < 0.05, least significant difference test).
The activity of the antioxidant system also obviously changed after the CNM treatments. The activities of the antioxidant enzymes SOD and POD significantly increased by almost 20% in the CNM treatment groups after 14 d of treatment (Fig. 3E and 3F), CAT activity significantly increased in the GQD group, and no significant difference was found between the C60 and distilled water groups (Fig. 3G). Regarding the nonenzymatic antioxidants, the AsA content was increased and GSH decreased slightly after the CNM treatments during the latter 3 stages. The AsA content peaked on the 7th d in the GQD and distilled water groups and on the 14th d in the C60 group (Fig. 3H). However, the GSH content peaked on the 21st d in the distilled water group and on the 7th d in the C60 group. The GSH content in the CNM treatments was significantly lower than that of the control after the 14th d of treatment (Fig. 3I). These results suggest that GQD and C60 affect the antioxidant system in carnation cut flowers.
Correlation analysis indicated that membrane lipid peroxidation products showed a significant positive correlation with H2O2 (p = 0.0326) and CAT (p = 0.0173) in the distilled water group (Table 2). Additionally, CAT activity displayed a significant positive correlation with H2O2 (p = 0.0447) and a significant negative correlation with O2·- (p = 0.0256); POD activity showed a significant positive correlation with OH· and a significant negative correlation with O2·-; and OH· displayed a significant negative correlation with O2·- (Table 2). These results suggest that excess O2·- can be transformed into H2O2 and OH· and that H2O2 is the major factor for membrane lipid peroxidation in cut flowers. CAT and POD can scavenge ROS components. Interestingly, ROS metabolism and the activity of the antioxidant system showed obvious changes in the CNM treatments. MDA showed a significant positive correlation with POD (p = 0.0001 and 0.0083) and OH· (p = 0.0276 and 0.0498) in the GQD and C60 groups, and POD was positively correlated with OH· (p = 0.0250 and 0.0441) (Table 2). Furthermore, O2·- had a significant negative correlation with OH· (p = 0.0107) in the GQD group (Table 2). These results indicated that GQD and C60 may influence ROS metabolism, whereby OH· and POD play important roles in cell damage and oxidative stress defense, respectively.
Table 2.
The correlation analysis of oxidative physiological indices of carnation cut flowers treated with distilled water, 25 mg·L-1 GOD and 1 mg·L-1 C60
Bold values indicate a significant correlation between the two indices. GQD: graphene quantum dots, C60: fullerene, MDA: malondialdehyde, SOD: superoxide dismutase, POD: peroxidase, CAT: catalase, O2·-: superoxide anion radical, H2O2: hydrogen peroxide, OH·: hydroxyl radical, AsA: ascorbic acid, GSH: glutathione. Each experiment was repeated 3 times. All the data are correlation coefficients, and significance levels are indicated at * p < 0.05 or ** p < 0.01.
Free Radical Scavenging Ability Test
The in vitro Fenton reaction system was employed to evaluate the OH· inhibition capacity of CNMs. Both C60 and GQD inhibited OH· generation by approximately 10% to 15%, and the inhibitory effect was gradually enhanced with increasing CNM concentration (Fig. 4A). The free radical scavenging ability was evaluated using the principle of single-electron transfer. The DPPH scavenging activity of GQD was increased with increasing concentration; however, there were no significant differences in the scavenging activity of C60 between the different concentrations (Fig. 4B). These results suggest that C60 and GQD might play a positive role in inhibiting OH· generation, and GQD may also be involved in regulating the redox status of plant cells.
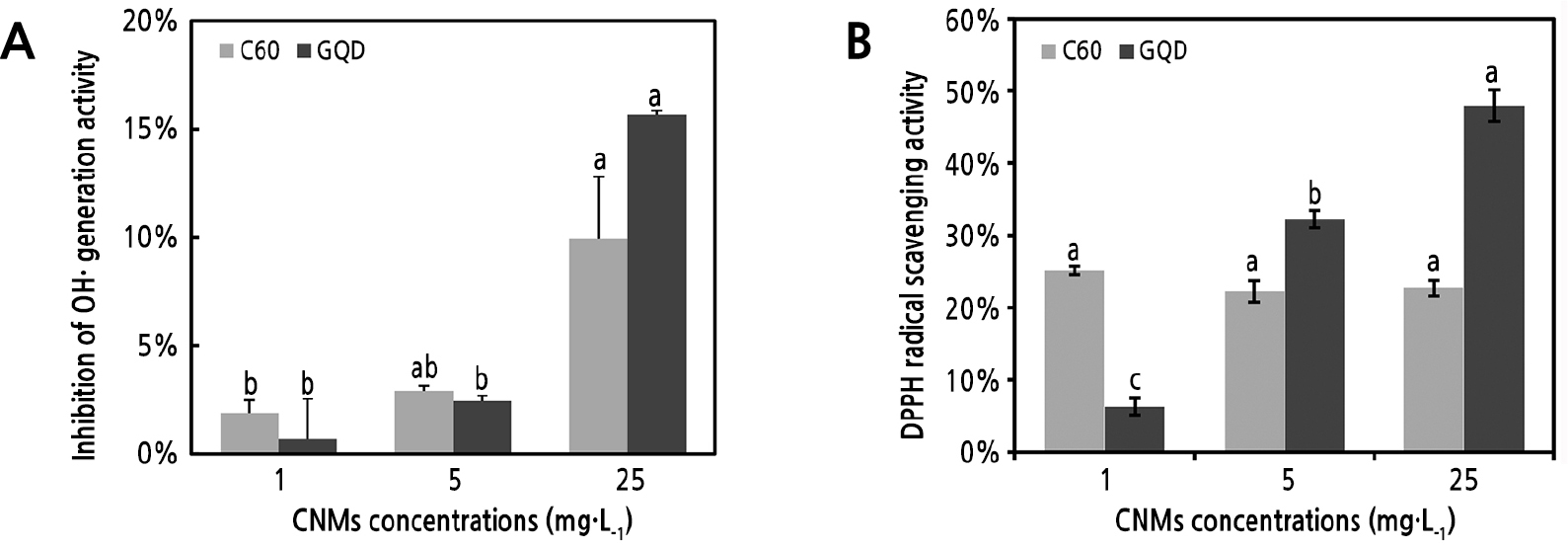
Fig. 4.
Inhibition of OH· generation and DPPH radical scavenging by CNMs. In vitro detection of Fenton reaction (A), and in vitro detection of DPPH radical scavenging activity (B). Each experiment was repeated 3 times, vertical bars represent standard error of the mean (± SE), and different letters represent significant differences (p < 0.05, least significant difference test) between different samples treated with the same CNM.
Discussion
In carnation, membrane deterioration and senescence are associated with a decrease in respiratory activity (Song et al., 2008). Vase solutions containing adenosine triphosphate (ATP) or spermidine can extend the VL of cut carnation flowers (Tassoni et al., 2006; Song et al., 2008). In this study, cut carnation flowers gradually deteriorated during the 3 weeks after harvest, and different CNMs had different effects on the VL of carnation. Lower concentrations of SWCNT and C60 extended the VL, but higher concentrations did not have this effect. By contrast, 25 mg·L-1 GQD was better able to inhibit senescence and abscission. Thus, the physiological functions of CNMs differed depending on their different properties and concentrations. SWCNTs affect root growth in different plants (Canas et al., 2008). Fullerenes can be easily taken up and translocated by plant roots due to their small size; however, CNTs only penetrate the epidermis and cannot be transported into the cortex and vascular tissue (Zuverza-Mena et al., 2017). Accordingly, we speculated that C60 and GQD can be absorbed and transported into plant tissues and perform their biological effects. SWCNT can not be transported into plant tissues, and higher concentrations of SWCNTs can block the vascular tissue of the stem, causing the RWC to significantly decrease.
Suitable concentrations of C60 and GQD can influence the ROS levels and the antioxidant system, improving the VL of carnation cut flowers. After GQD and C60 treatments, the MDA and OH· levels of plant cells decreased significantly, and the main factors that cause cell damage were changed from H2O2 to OH·. OH·, the most active ROS, can attack all macromolecules and most cell constituents. Because of the absence of any enzymatic reaction to eliminate OH·, excess accumulation of OH· ultimately leads to cell death (Tanoua et al., 2009). Furthermore, in vitro inhibition of OH· and the DPPH free radical scavenging assay suggested that C60 and GQD can inhibit OH· generation and maintain the redox state of cells. Many studies have reported that CNMs influence plant growth, development, and stress tolerance (Liu et al., 2017). Anjum et al. (2013, 2014) found that graphene oxide (GO) increased electrolyte leakage, the H2O2 content, and lipid and protein oxidation in broad bean (Vicia faba). He et al. (2018) reported that low doses of GO (0.1 mg·L-1) can inhibit microbial growth and improve water uptake and the VL of cut roses (Rosa sp.). Fullerenes and their derivatives have been ascribed to suppress the accumulation of O2·- and OH·, which are involved in lipid peroxidation. The seed yield of bitter melon (Momordica charantia) increased by 112% to 128% after treatment with fullerene; however, the effect diminished with a high concentration of C60 (Husenl and Siddiqi, 2014). Fullerenes (С60 and С70) can penetrate cell membranes and influence growth, crop yield, and relieve oxidative stress in barley and wheat (Triticum aestivum) (Panova et al., 2018). Chen et al. (2004) reported that C60(OH) significantly suppressed ROS production and reversed the decrease of mitochondrial membrane potential induced by H2O2. However, a high concentration of C60(OH) (1 and 1.5 mM) lead to cell death. Karimi et al. (2012) treated carnation with aminooxyacetic acid, benzyladenine, and 1-methylcyclopropene, which significantly decreased the concentration of H2O2 and superoxide radicals, and significantly increased the activities of SOD, CAT, and POD compared with the untreated control, and these treatments can be suitable for extending the longevity of cut carnation flowers. Floral bud abscission is regulated by ethylene and endogenous sugars, and can be prevented by treatments with inhibitors of the ethylene receptor or sugars (van Doorn and Han, 2011). Postharvest physiological studies should be carried out to further verify whether CNMs indirectly affect the levels of endogenous hormones and carbohydrate metabolism to delay the senescence of cut flowers. Our study found that C60 and GQD can directly affect intracellular ROS metabolism, resulting in changes in the cell redox state, an increase in the activity of the antioxidant system, and reduced membrane lipid peroxidation, thereby delaying plant tissue senescence.
Conclusions
C60 and GQD can extend the VL of carnation cut flowers by approximately 10%, and higher concentrations of SWCNT can block vascular tissues and decrease the VL. C60 and GQD may inhibit OH· generation by approximately 10% to 15%, and GQD had higher DPPH free radical scavenging capacity. Accordingly, suitable concentrations of C60 and GQD can influence ROS metabolism and downstream biological events, including the cell redox state, the antioxidant system, and membrane lipid peroxidation, resulting in delayed plant tissue senescence (Fig. 5).