Introduction
Materials and Methods
Plant Materials, Growth Conditions and Seedling Treatments
Vector Construction of SlPIP1;7 and Tomato Transformation
Determination of Biomass
Measurement of Photosynthetic Parameters
Water Potential and Hydraulic Conductance
Determination of the Relative Electrolyte Leakage and MDA
Determination and Histochemical Detection of ROS
Statistical Analysis
Results
Phenotypes of Wild-type and Transgenic Plants
Morphological Changes under Salt Stress
Photosynthesis of the Different Tomato Lines under Salt Stress
The Water Potential and Hydraulic Conductivity of Tomato Plants under Salt Stress
Accumulation of ROS in Various Tomato Plants under Salt Stress
Detection of Cell Membrane Injury of the Different Tomato Lines under Salt Stress
Discussion
Introduction
Soil salinity can greatly affect plant growth by causing osmotic stress and ion toxicity, and results in major crop yield losses worldwide (Zhu et al., 2020). Ion toxicity causes ionic imbalance in plants, leading to leaf necrosis and even death, while osmotic stress inhibits root water uptake by reducing root hydraulic conductivity (Lpr) (Qian et al., 2015). Lpr can be decreased by salt, drought, and other abiotic stresses (Sánchez-Romera et al., 2014). Decreased Lpr under salt stress conditions may slow water flow from the roots to the soil when the soil has a lower osmotic potential than the roots (Aroca et al., 2012). Previous studies have shown that water absorption under salt stress was largely dependent on the amount and activity of aquaporin (AQPs), which could be altered by changes of Lpr (Han et al., 2020a).
AQPs are a class of water-channel proteins that selectively and efficiently mediate the transport of water across membranes, and are widely distributed in plant roots, stems, leaves, seeds, and floral organs (Verdoucq and Maurel, 2018). AQPs are usually classified into seven subfamilies, including plasma-membrane intrinsic proteins (PIPs), tonoplast intrinsic proteins (TIPs), nodulin-26-like intrinsic proteins (NIPs), hybrid intrinsic proteins (HIPs), GlpF-like intrinsic proteins (GIPs), small basic intrinsic proteins (SIPs) and X intrinsic proteins (XIPs) (Maurel et al., 2015). Proteins in the PIP subfamily, which is one of the most extensively studied groups, mediate two-way transport of water and neutral molecules across the membrane (Maurel et al., 2016). PIPs play vital roles in maintaining the water balance in plants under stress conditions (e.g., salt, drought, abnormal temperature, high CO2 concentration, or nutrient deficiency). Plants can adapt to such stress by regulating the expression of PIPs, thus affecting Lpr (Kapilan et al., 2018; Jia et al., 2020). A previous study reported that Lpr and PIP expression in barley (Hordeum vulgare) roots decreased significantly under salt stress (Przedpelska-Wasowicz and Wierzbicka, 2011). Pou et al. (2016) observed that Lpr in roots of Arabidopsis thaliana under salt stress decreased with decreasing promoter activity and PIP2;7 transcript level. However, contrary results regarding the Lpr and PIP expression under various abiotic stresses have also been reported. For example, the transcripts of most PIPs in maize (Zea mays) were up-regulated under drought stress, while the Lpr decreased (Hachez et al., 2012). Similarly, salt treatment increased the transcripts of most PIP genes of Arabidopsis, except PIP2;6 and PIP1;5 in roots (Jang et al., 2004). Additionally, root Lpr in Arabidopsis decreased rapidly in response to 45 min of salt stress, while the transcript levels of most PIPs and TIPs were down-regulated after 4 h of salt stress (Boursiac et al., 2005). These studies demonstrated that the expression of PIPs varies depending on the tissues, plants, and stress conditions, and some PIPs exhibit different responses to the same stress condition. Moreover, there was evidence that PIPs played important roles in plant photosynthesis, since the overexpression of NtAQP1 in tobacco (Nicotiana tabacum) increased the photosynthetic rate (Pn) by 20% compared to controls (Flexas et al., 2006).
Cultivated tomato (Solanum lycopersicum), one of the most popular fruit in the world, has moderate sensitivity to soil salinity, which makes it a useful model plant for studying the functions of genes related to salt stress (Han et al., 2020a). The tomato AQP family consists of 47 members, including 14 SlPIPs (Reuscher et al., 2013). However, the functions of most of these PIP isoforms under abiotic stresses are still unknown. In our previous study, the SlPIP1;7 gene showed differential expression profiles in response to salt stress in tomato leaves and roots. Salt stress rapidly up-regulated the expression of SlPIP1;7 after 1 h; however, its expression decreased after 24 h of salt stress, but it was fully or partially recovered after being transferred to the control culture solution (Jia et al., 2020). Furthermore, among various SlPIP isoforms, SlPIP1;7 exhibited relatively high expression in the roots of a wild tomato species (Solanum pimpinellifolium ‘L03708’) compared to a cultivated tomato (‘M82’) under salt stress (data not shown). These results imply that SlPIP1;7 may play an important role in regulating water transport under salt stress conditions. To determine the function of SlPIP1;7 under salt stress, we generated SlPIP1;7-overexpressing and SlPIP1;7-silenced transgenic tomato lines. We investigated the Lpr, reactive oxygen species (ROS) accumulation, electrolyte leakage and malondialdehyde (MDA) content in wild-type and transgenic plants under salt stress. This study sheds light on the role of SlPIP1;7 in water transport in tomato plants under salt stress, and reveals that SlPIP1;7 is a valuable genetic resource for breeding salt-tolerant tomato plants.
Materials and Methods
Plant Materials, Growth Conditions and Seedling Treatments
The seeds of tomato (Solanum lycopersicum L.) cultivar ‘AC’ were sterilized in a water bath at 55°C for 15 min and cultured in an incubator at 28°C for 2 days. The germinated seeds were sown in a commix medium in a glasshouse. Three-leaf seedlings were transferred to containers filled with 1/4 strength of Hoagland nutrient solution, then transferred to 1/2 strength of Hoagland nutrient solution after 3 days (Jia et al., 2020). For salt stress treatment, 150 mM NaCl was added to the nutrient solution. Meanwhile, the plants grown in the nutrient solution without addition of NaCl were used as control. All plants were grown in the glasshouse under natural light with day/night temperatures of 28°C/18°C. The plant samples were collected and quickly frozen in liquid nitrogen prior to being stored at −80°C for further analysis.
Vector Construction of SlPIP1;7 and Tomato Transformation
The SlPIP1;7 gene (GenBank accession no. NP_001289851) coding sequence was obtained by RT-PCR amplification from total RNA isolated from tomato leaves with the RevertAid First Stand cDNA Synthesis Kit and the PCR amplification kit (Thermo Scientific, USA). To construct the plant overexpression vector, forward (5'-GCTCTAGAATGGAGCATA GAGAAGAGG-3') and reverse (5'-GGGGTACCTCATTTGGACTTGAATGGG-3') primers were designed to amplify the complete ORF of SlPIP1;7 and inserted into the pMBP1 plasmid at XbaI and KpnI endonuclease sites. To generate the RNAi construct, two 217-bp fragments of SlPIP1;7 were amplified with primers that included restriction sites for XbaI/HindIII and XhoI/KpnI, respectively (SlPIP1;7-1F: 5’-GCTCTAGAACATTTGGGCTTTTCTTAGCG-3’; SlPIP1;7-1R: 5’-CCCAAGCTTAGACAAGAACAAATGTGCCGAT-3’; SlPIP1;7-2F, 5’-CCGCTCGAG ACATTTGGGCTTTTC TTAGCG-3’; SlPIP1;7-2R, 5’-GGGGTACCAGACAAGAACAAATGTGCCGAT-3’). The amplified fragment products were then digested and inserted into a pKANNIBAL vector and then digested with NotI and ultimately inserted into the binary plasmid pART27 (Wesley et al., 2001). The PMBP1 and two recombinant vectors were subsequently transferred into Agrobacterium tumefaciens strain LBA4404 with the leaf disc method through callus, shoot, and root induction (Han et al., 2020b). Three independent overexpression lines and two RNAi transformants (T2 generation) were obtained after selection on kanamycin plates and were labeled as OE1, OE4, OE7, RNAi3, and RNAi8, respectively.
Determination of Biomass
The plant height (from the base of the stem to the highest growth point of the stem), the second internode length (from the second to the third fully developed leaf) and the stem diameter (the middle part of the plant) of tomato plants were measured by a straight ruler and vernier caliper. For each replication, at least 10 seedlings were measured.
Measurement of Photosynthetic Parameters
The net photosynthesis rate (Pn), stomatal conductance (Gs), transpiration rate (Tr), and cellular CO2 concentration (Ci) were detected with a portable photosynthesis system (Li-6800; LI-COR Inc., Lincoln, NE, USA). The second fully expanded leaf was assayed at a photon flux density of 800 µmol m-2·s-1. Six biological replicates were used for these experiments.
Water Potential and Hydraulic Conductance
The water potential of leaf and root was assayed with a pressure chamber (PMS Model 1505D, USA). The second fully expanded leaves were used to determine the leaf water potential according to Zhu et al. (2015). The hydraulic conductance of leaves (Kleaf) and roots (Lpr) was measured using the same pressure chamber (Han et al., 2020a). The root and leaf surface area was determined using a root scanner (Model MRS-9600TFU2L, Microtek, Shanghai, China). The hydraulic conductance was calculated according to Han et al. (2020a). Three biological replicates were used for these experiments.
Determination of the Relative Electrolyte Leakage and MDA
The leaf electrolyte leakage was assayed after salt stress for various periods of time according to Han et al. (2020b). The concentration of MDA was determined with the thiobarbituric acid reaction (Shi et al., 2014).
Determination and Histochemical Detection of ROS
The content of hydrogen peroxide (H2O2) and superoxide anion (O2−) in the leaves was tested following the method of Han et al. (2020b) and Puyang et al. (2015), respectively. The content of H2O2 and O2− was calculated with a standard curve. The in situ formation of H2O2 and O2− in the plants was detected by dipping the leaves into diaminobenzidine (DAB) and nitroblue tetrazolium (NBT), respectively (Han et al., 2020b). Six biological replicates were used for these experiments.
Statistical Analysis
The data were subjected to Student’ s t-test using SPSS 19.0 software (IBM, Armonk, NY).
Results
Phenotypes of Wild-type and Transgenic Plants
To investigate the function of SlPIP1;7 in tomato plants, we constructed transgenic tomato plants in which SlPIP1;7 was overexpressed or silenced (Fig. 1). Three SlPIP1;7-overexpressing lines (OE-1, OE-4 and OE-7) and two SlPIP1;7-silenced transgenic lines (RNAi-3 and RNAi-8) were obtained (Fig. 1A, and 1B). SlPIP1;7-overexpressing and -silenced plants were taller and shorter than wild-type plants, respectively (Fig. 1C). The silenced lines had significantly lower internode length and stem diameter than the wild-type and SlPIP1;7-overexpressing plants. These results indicated that overexpression of SlPIP1;7 in the transgenic lines enhanced tomato growth relative to that of the wild-type, while the shoot and root growth of SlPIP1;7-silenced seedlings were severely retarded.
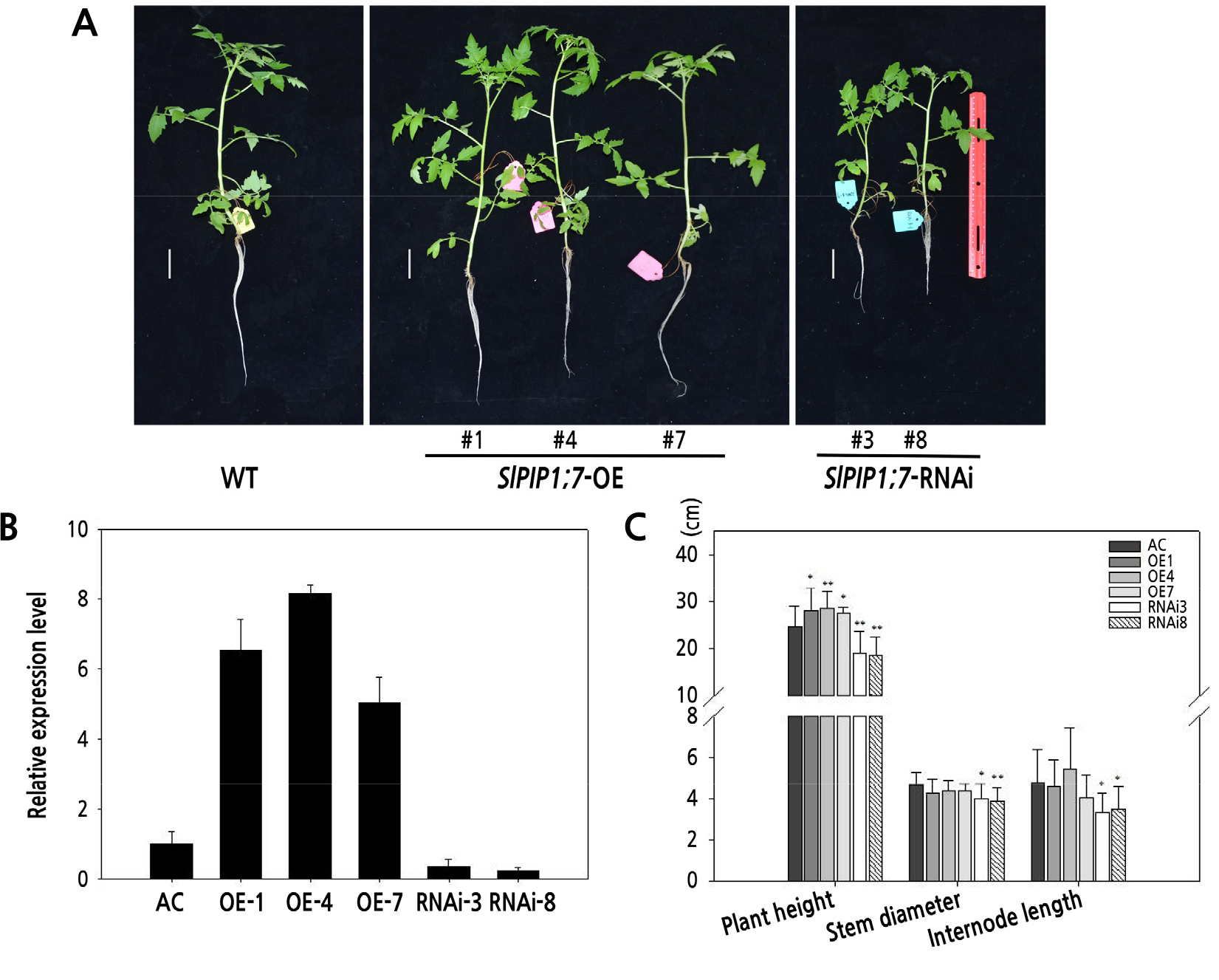
Fig. 1.
Comparison of plant growth among WT and SlPIP1;7 transgenic plants. (A) Phenotypic characterization of six-week-old wild-type and SlPIP1;7 transgenic plants grown in hydroponic culture. Bars = 5 cm. (B) Relative expression levels of SlPIP1;7 in the leaf tissues of SlPIP1;7-overexpressing and SlPIP1;7-silenced lines. Data are represented as mean ± SD (n = three biological replicate samples). Each replicate sample consisted of leaves from three seedlings. (C) Plant height, stem diameter and internode length of wild-type and SlPIP1;7 transgenic plants. The statistical significance was estimated by a Student’ s t-test (n > 10). *, p < 0.05; **, p < 0.01. Error bars represent ± SD.
Morphological Changes under Salt Stress
To explore the role of SlPIP1;7 in the salt stress response, wild-type and SlPIP1;7 transgenic plants (T2 generation) were treated with 150 mM NaCl for different periods of time (Fig. 2). After treatment for 24 h, SlPIP1;7-overexpressing plants showed little change, while the wild-type showed slight withering and the SlPIP1;7-silenced seedlings showed severe wilting.
Photosynthesis of the Different Tomato Lines under Salt Stress
The net photosynthesis rate (Pn) was measured in wild-type and SlPIP1;7 transgenic plants under salt stress for different periods of time. SlPIP1;7-overexpressing lines had significantly higher Pn than wild-type plants both under control and salt stress conditions (Table 1). Salt stress treatment for 6 h significantly reduced the Pn of wild-type and SlPIP1;7-overexpressing tomato leaves (33.8% and 16.9%, respectively, vs. the control condition). SlPIP1;7-silenced plants had significantly lower Pn than wild-type plants under normal conditions, and after NaCl treatment for 24 h. Changes in the stomatal conductance (Gs) of SlPIP1;7-overexpressing lines were consistent with changes in Pn. The transpiration rate (Tr) in SlPIP1;7-overexpressing lines was two times higher than that of the control plants after salt stress for 24 h. Salt stress treatment significantly reduced the intracellular CO2 concentration (Ci) of wild-type and SlPIP1;7 transgenic tomato leaves; however, Ci was still higher in SlPIP1;7-overexpressing lines than in wild-type and SlPIP1;7-silenced plants.
Table 1.
The net photosynthetic rate (Pn), transpiration rate (Tr), stomatal conductance (Gs), and intracelluar CO2 (Ci) of WT, SlPIP1;7-overexpressing and SlPIP1;7-silenced tomato plants under salt stress. OE and RNAi represent three SlPIP1;7-overexpressing lines and two SlPIP1;7-silenced lines, respectively
The Water Potential and Hydraulic Conductivity of Tomato Plants under Salt Stress
Water potential has been used as an index of the water status, and salt and drought stress, of crops in arid regions. In this study, the SlPIP1;7-overexpressing plants showed significantly higher leaf and root water potential than wild-type plants under normal conditions. SlPIP1;7-overexpressing plants still had higher root water potential than wild-type plants after salt stress treatment for 6 h and 24 h, as well as elevated leaf water potential after salt stress treatment for 12 h (Fig. 3A and 3B). The water uptake and capabilities of plants can be expressed by Lpr and leaf hydraulic conductivity (Kleaf). To investigate the effect of salt stress on water status, we measured the Kleaf and Lpr of wild-type and transgenic tomato seedlings under normal and salt stress conditions (Fig. 3C and 3D). Kleaf and Lpr did not differ significantly among wild-type and transgenic plants under normal conditions. However, the Kleaf and Lpr of SlPIP1;7-overexpressing plants increased significantly after salt treatment for 6 h compared to wild-type and SlPIP1;7-silenced plants. In addition, no significant difference in Lpr was observed between wild-type and SlPIP1;7-silenced plants, but Kleaf decreased significantly in SlPIP1;7-silenced plants after salt treatment for 24 h compared to wild-type and SlPIP1;7-overexpressing plants.
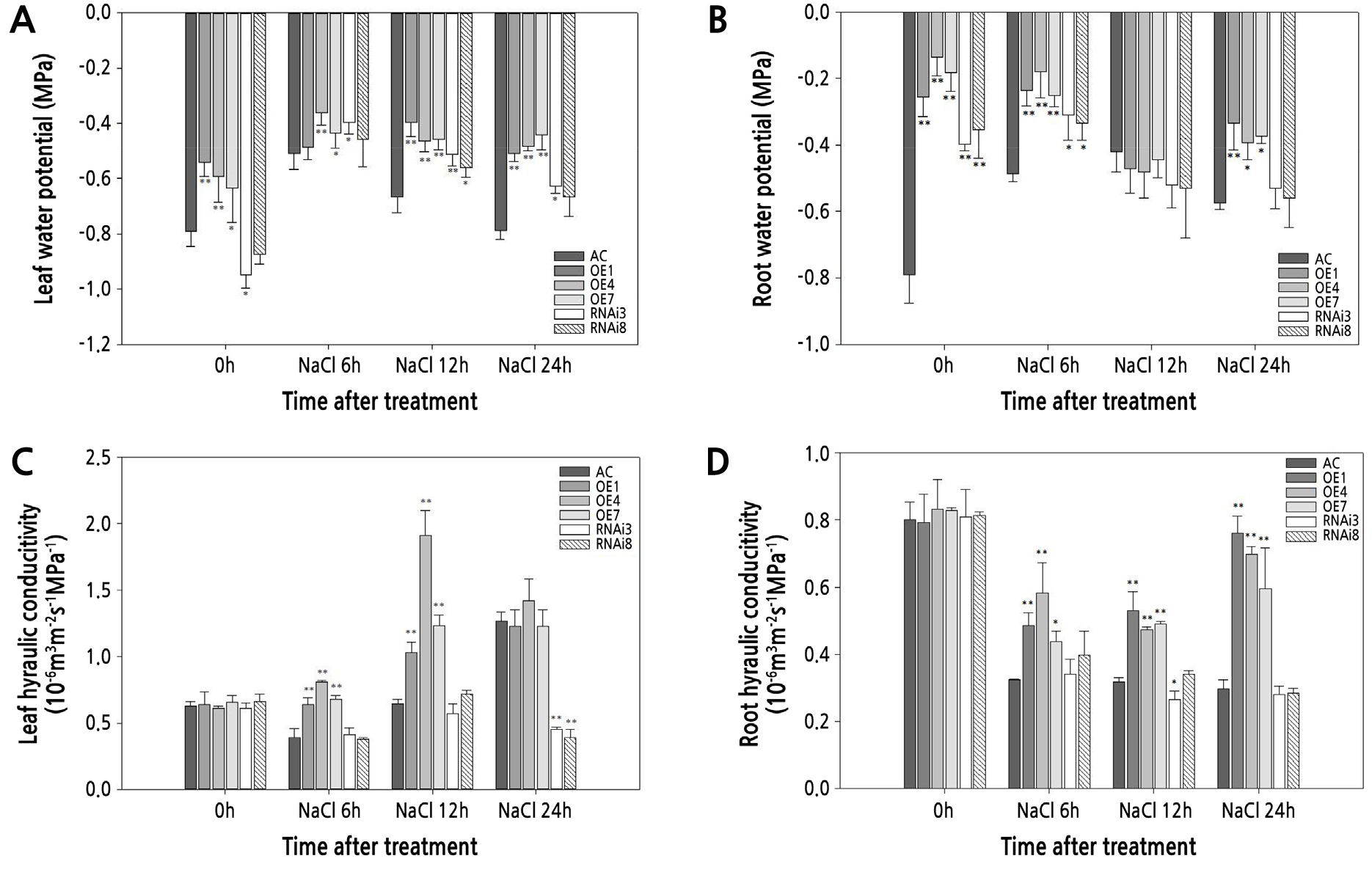
Fig. 3.
Effect of salt stress on water potential and hydraulic conductance of tomato seedlings. (A) Leaf and (B) root water potential under salt treatment for various periods of time. (C) Leaf (Kleaf) and (D) root hydraulic conductivity (Lpr) under salt treatment for various periods of time. The statistical significance was estimated by a Student’ s t-test (n > 3). *, p < 0.05; **, p < 0.01. Error bars represent ± SD.
Accumulation of ROS in Various Tomato Plants under Salt Stress
Hydrogen peroxide (H2O2) and superoxide anion (O2−) accumulation in leaves under normal and salt stress conditions were evaluated (Fig. 4). H2O2 and O2− contents did not differ significantly among the tomato lines under control conditions. After salt stress treatment, all the tomato plants showed increased staining, indicating that salt stress increased the accumulation of ROS in all of the plant types (Fig. 4A and 4B). In addition, the accumulation of H2O2 and O2− was lower in SlPIP1;7-overexpressing plants than in wild-type plants under salt stress treatment. The accumulation of O2− in SlPIP1;7-silenced plants was significantly higher than that in wild-type plants. H2O2 contents in SlPIP1;7-silenced plants were higher after 6 h of salt stress treatment, but lower after 24 h, compared to wild-type plants. The H2O2 and O2− contents of leaves in wild-type and transgenic plants under normal and salt stress conditions (Fig. 4C and 4D) were consistent with the staining results.
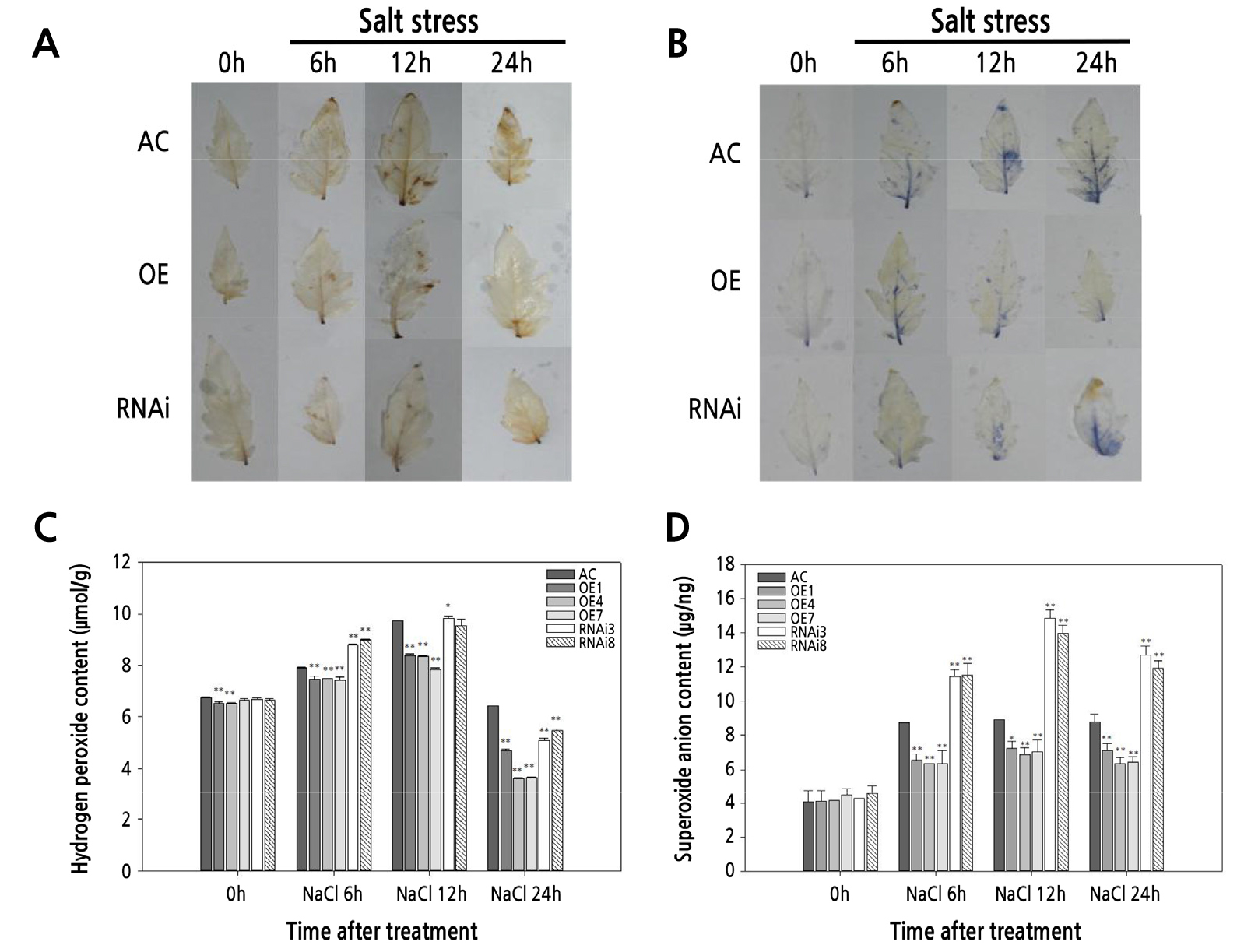
Fig. 4.
Comparison of reactive oxygen species (ROS) accumulation in leaves of WT and SlPIP1;7-transgenic plants under salt stress at various time points. (A) Diaminobenzidine (DAB) staining of hydrogen peroxide (H2O2) accumulation in leaves. (B) Nitroblue tetrazolium (NBT) staining of superoxide anion (O2−) accumulation in leaves. Comparison of the content of H2O2 (C) and O2− (D) in leaves of transgenic and wild-type plants under NaCl treatment. The statistical significance was estimated by a Student’ s t-test (n > 3). *, p < 0.05; **, p < 0.01. Error bars represent ± SD.
Detection of Cell Membrane Injury of the Different Tomato Lines under Salt Stress
The cell membrane damage in the tomato lines under salt stress was assessed by detecting the relative levels of electrolyte leakage and MDA accumulation (Fig. 5). There was no significant difference in electrolyte leakage among SlPIP1;7-overexpressing and wild-type plants; however, leaf electrolyte leakage increased significantly in SlPIP1;7-silenced plants after salt stress (Fig. 5A). MDA content did not differ significantly among the tomato lines under control conditions (Fig. 5B). SlPIP1;7-overexpressing plants had a lower MDA content than SlPIP1;7-silenced and wild-type plants, regardless of the length of the salt stress treatment. The MDA content in SlPIP1;7-silenced plants was significantly higher than that of the wild-type and SlPIP1;7-overexpressing plants after salt stress.
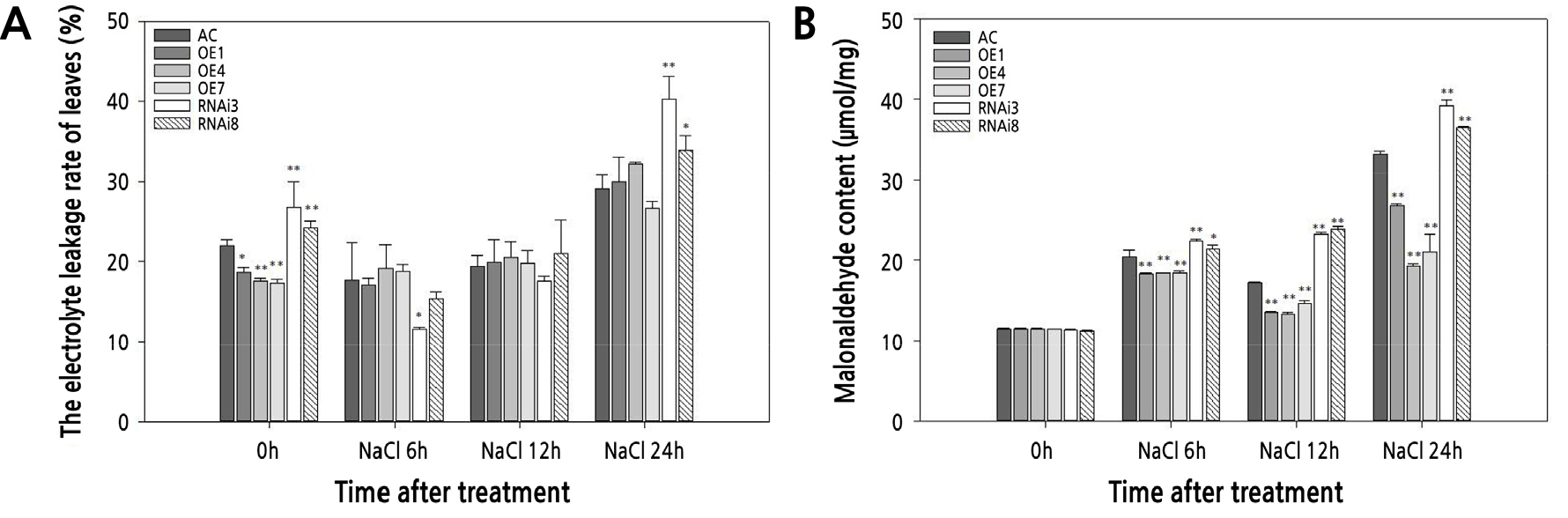
Fig. 5.
Comparison of the electrolyte leakage rate and malondialdehyde (MDA) accumulation in leaves of WT and SlPIP1;7-transgenic plants under salt stress at various time points. (A) The relative electrolyte leakage of tomato leaves from WT and SlPIP1;7 transgenic tomato plants. (B) The MDA content of tomato leaves from WT and SlPIP1;7 transgenic tomato plants. The statistical significance was estimated by a Student’s t-test (n > 3). *, p < 0.05; **, p < 0.01. Error bars represent ± SD.
Discussion
PIPs were the first class of AQPs to be defined, and most currently known AQPs are PIPs (Maurel et al., 2016). PIPs are the main pathway of water transport through cellular membranes and play a vital role in water absorption by plants (Maurel et al., 2015). PIPs are divided into PIP1 and PIP2 subclasses in higher plants, according to their characteristics and N-terminal and C-terminal activities (Maurel et al., 2016). PIP1s are distributed in almost all tissues, which suggests that they may play multiple roles in plant growth and development (Biela et al., 1999). Previous studies have shown that PIP1s are involved in growth and development processes of plants, such as seed maturation and germination, lateral root development, flowering, and fruit maturation (Gattolin et al., 2011; Reinhardt et al., 2016; Kong et al., 2018). Furthermore, some studies have shown that PIP1s can improve the growth rate, Pn, and water use efficiency of plants (Sade et al., 2010; Pawlowicz and Masajada, 2019). In our study, overexpression of SlPIP1;7 in the transgenic line enhanced tomato plant growth compared with wild-type plants, while the growth of SlPIP1;7-silenced seedlings was suppressed (Fig. 1A and 1C). Moreover, SlPIP1;7-overexpressing plants had significantly higher Pn, Gs, Tr, and leaf water potential than wild-type plants under control conditions (Table 1). Tomato plant Ci was significantly reduced by salt stress treatment, but it was higher in SlPIP1;7-overexpressing lines than in wild-type and SlPIP1;7-silenced plants. The stomatal opening and transpiration of most crops decreases under salt stress, which leads to insufficient CO2 supply, resulting in a decline in Pn. Hence, Ci reflects CO2 supply capacity and the potential utilization of CO2 by photosynthetic organs (Xu et al., 2016). The overexpression of NtAQP1 in tobacco increased Pn and Ci of plants under normal growth conditions, which yielded a major improvement in productivity due to the high photosynthetic rate (Sade et al., 2010). In our study, we found that the overexpression of SlPIP1;7 in transgenic lines increased Gs and improved the leaf water status under control conditions, thus enhancing photosynthesis and Tr, and further improving plant growth and development compared to wild-type plants. The decrease in these photosynthetic indices may also have restricted the growth of the SlPIP1;7-silenced plants. Furthermore, overexpression of SlPIP1;7 has been reported to increase plant Lpr, suggesting that increasing membrane permeability might be an important role of SlPIP1;7 in its impact on the whole plant (Fig. 2). Thus, SlPIP1;7 has a dual impact not only at the cellular level but also at the whole-plant level, where its expression increases both transpiration and net photosynthetic fluxes.
Salt stress treatment for 6 h significantly reduced the Pn of wild-type and SlPIP1;7-overexpressing tomato leaves, by 33.8% and 16.9%, respectively, compared to the control condition (Table 1). Additionally, the Kleaf of SlPIP1;7-overexpressing plants increased significantly compared to wild-type and SlPIP1;7-silenced plants after salt stress treatment for 6 h (Fig. 2). Kleaf is a major determinant of Pn in plants under both optimal and adverse environmental conditions (Scoffoni et al., 2012). In general, overexpression of SlPIP1;7 attenuated the decreased Pn in tomato leaves caused by salt stress, which might help explain the increased salt tolerance of SlPIP1;7-overexpressing plants. Similarly, a previous study reported that overexpression of NtAQP1 in tobacco improved Pn, leaf CO2 conductivity, Gs, and water use efficiency under both control and salt stress conditions (Sade et al., 2010). Overexpression of the barley HvPIP2;1 isoform in rice (Oryza sativa) plants was found to increase Pn (Hanba et al., 2004). Another study found that transgenic tomato plants overexpressing TIP2;2 were more resistant to drought and salt stress than controls and had improved yield under control and stress conditions, since a high level of TIP2;2 expression increased transpiration under normal growth conditions and limited the reduction of transpiration under drought and salt stress conditions (Sade et al., 2009).
Environmental stresses, such as salinity and drought, usually inhibit root water uptake and alter water balance, thus limiting the growth and development of plants (Gong et al., 2014). The water uptake capabilities of plants can be expressed in terms of Kleaf and Lpr, which are important indicators of a plant’s ability to cope with abiotic stress (Qian et al., 2015). Soil salinity, a kind of osmotic stress, usually inhibits root water absorption and affects the expression of AQPs, which in turn affects Lpr (Han et al., 2020a). It was reported that Lpr in Arabidopsis decreased as the transcript level of PIP2;7 decreased under salt stress (Pou et al., 2016). In our study, overexpression of SlPIP1;7 in tomato plants improved their tolerance to salt stress treatment, whereas SlPIP1;7-silenced plants were more sensitive to salt stress. Furthermore, the root Lpr of SlPIP1;7-overexpressing plants increased after salt treatment for 6 h compared to wild-type and SlPIP1;7-silenced plants (Fig. 2). These results suggest that tomato SlPIP1;7 protein may increase the resistance of plants to salt stress, at least in part, by regulating the early hydraulic conductivity and maintaining water potential in roots. Increasing Gs and photosynthetic efficiency while maintaining normal root Lpr under salt stress conditions suggests that SlPIP1;7 has two independent roles in whole-plant hydraulic control.
Plants continuously generate ROS under favorable conditions, which is a product of multiple metabolic pathways (Das and Roychoudhury, 2014). A threshold level of ROS is required for normal physiological processes like signal transduction, cell growth, and activation of endogenous antioxidants (Banerjee et al., 2020). Salt stress increases the accumulation of ROS, such as H2O2, O2-, and O•−, which can induce oxidative damage in plants, potentially leading to cytoplasmic membrane damage and even cell death (Ahanger et al., 2019). In addition, MDA accumulation and relative electrolyte leakage reflect the degree of membrane damage of plants under abiotic stress (Han et al., 2020b). SlPIP1;7-overexpressing plants under salt stress accumulated significantly less H2O2 and O2− than wild-type plants, while SlPIP1;7-silenced plants accumulated more (Fig. 3). Furthermore, SlPIP1;7-overexpressing plants had lower MDA content than SlPIP1;7-silenced and wild-type plants, and SlPIP1;7-silenced plants showed significantly more electrolyte leakage and MDA accumulation than wild-type plants under salt stress. Thus, SlPIP1;7-overexpressing plants had a strong antioxidant defense.
In the present study, we created transgenic tomato plants in which SlPIP1;7 was overexpressed or silenced. The overexpression of SlPIP1;7 increased Gs and improved leaf water status under control conditions, thus enhancing Pn and further improving the growth and development of SlPIP1;7-overexpressing plants compared to wild-type plants. Moreover, SlPIP1;7-overexpressing plants were tolerant to salt stress, while SlPIP1;7-silenced plants were more sensitive. The SlPIP1;7 protein may increase the resistance of tomato plants to salt stress by increasing the Gs and Kleaf of leaves, resulting in increased photosynthetic efficiency, and by maintaining normal Lpr and promoting root water uptake under salt stress conditions (Fig. 6). SlPIP1;7-overexpressing plants exhibited less plasma membrane damage, better maintenance of plasma-membrane integrity, and stronger oxidation resistance. These results elucidate the relationship between plant salt tolerance and SlPIP1;7, which may be a useful biotechnological tool for improving the salt tolerance of different plant species.