Introduction
Materials and Methods
Plant Materials and Treatment
Anatomical Study of Inflorescence Bud Development
Inflorescence Trait Data Collection
Estimation of Pollen Viability
Estimation of Pollen Germination in the Pistil
Determination of Pollen Enzyme Activities
Estimation of Fruit Set
Statistical Analysis
Results and Discussion
Inflorescence Bud Development
Inflorescence Traits
Pollen Viability
Pollen Germination in the Pistil
Pollen Enzyme Activities
Fruit Set
Conclusion
Introduction
Brassinosteroids (BRs) are natural plant growth regulators that are a type of plant steroid. BRs have been widely used for regulating various plant growth and development processes, including cell division and expansion, etiolation, reproduction, and abiotic stress tolerance (Szekeres et al., 1996; Thussagunpanit et al., 2013; Planas-Riverola et al., 2019). BRs play a critical role in plant reproductive functions, as they are essential for male fertility. They promoted pollen germination and tube growth in Arabidopsis (Vogler et al., 2014). BRs have been shown to initiate and maintain the tapetum layer during pollen development (Li and He, 2020) and to promote microspore embryogenesis (Ferrie et al., 2005). BRs modulate floral development and organogenesis by signaling with auxin and cytokinin (Kanwar et al., 2022). They are divided into many types according to their chemical structure. One BR that is generally used in agriculture is 24-epibrassinolide (EBR). However, it has the disadvantage of being expensive to synthesize and isolate. BR mimics that are more affordable have been synthesized with a chemical structure similar to that of a natural BR (Khamsuk et al., 2018). Specifically, 7,8-dihydro-8α-20-hydroxyecdysone (DHECD) is a BR mimic modified from 20-hydroxyecdysone in Vitex glabrata extract (Suksamrarn et al., 2002). DHECD has been shown to have functions similar to those of natural BRs in a number of ways (Thussagunpanit et al., 2017). Recent studies of DHECD have focused on mitigating the effect of abiotic stress in plants. For instance, the application of DHECD was shown to increase the efficiency of photosynthesis in cherry tomatoes (Maneechote et al., 2020) and to maintain membrane integrity in chili peppers under drought stress (Khamsuk et al., 2018). It can also maintain the rate of photosynthesis, antioxidative enzyme activities, and increase seed set levels under heat stress in rice (Thussagunpanit et al., 2015; Sonjaroon et al., 2018). However, to the best of our knowledge, there have been only limited reports on BR mimics when applied to fruit trees under normal seasonal production. Therefore, more study of the effects of BR and DHECD on fruit trees are needed to evaluate whether DHECD would be an effective BR mimic feasible for broad application to regulate fruit tree reproductive physiology.
The ‘Nam Dok Mai’ mango (Mangifera indica L.) is a well-known mango cultivar in Thailand. It has been exported to many countries and has been a strong source of revenue for the country. ‘Nam Dok Mai' mango growers are currently experiencing reductions in fruit set levels. Fertilization failures and infertile pollen are the main defects caused by an unfavorable climate during mango cultivation. Attempts have been undertaken to promote mango fruit set in a variety of ways, including the use of cross-compatible pollinizers, de-blossoming and the application of plant growth regulators. The application of auxin (NAA) and gibberellin (GA3) at the full-bloom stage can increase the percentage of fruit set in ‘Keitt’ mango (Nkansah et al., 2012). Polyamines have been reported to increase fruit set in ‘Dusheri’ and ‘Langra’ mangoes (Singh and Singh, 1995). In addition to these plant growth regulators, BRs enhance fruit set through male reproductive improvements. Therefore, it is of interest to use BRs and BR mimics to increase fruit set in ‘Nam Dok Mai’ mango. It has been hypothesized that exogenous BRs may be an effective solution to deal with the low fruit set problem in mangoes. Therefore, the present study elucidates the roles of EBR and DHECD on inflorescence development and pollen fertility. Furthermore, the most effective concentrations were determined for these BRs when applied to mangoes.
Materials and Methods
Plant Materials and Treatment
The study was conducted using five-year-old mango trees (Mangifera indica cv. Nam Dok Mai), located in Suphan Buri province (14°30'20.3"N 100°10'08.9"E) of Thailand. Flowering on the trees was managed according to standard farming practices. On the day the terminal bud developed to the ‘large bud swell’ stage (LB) (Oosthuyse, 1991), as indicated by a dome-shaped bud covered with green or brownish scales less than 3 cm in length, the buds were sprayed with two types of brassinosteroids, in this case EBR or DHECD. EBR (Ruina International Co. Ltd., China) and DHECD (Suksamrarn et al., 2002) were each sprayed at 0.01 or 0.1 µM concentrations. The control group was sprayed with water. The labeled buds were separately sprayed with approximately 5 mL of the BR solution for each treatment at 8.00–9.00 a.m. The data collected in this study are displayed in Fig. 1.
Anatomical Study of Inflorescence Bud Development
Inflorescence buds were collected four times at 2, 4, 8, and 12 days after BR application. The buds were fixed in formalin-acetic acid-alcohol (FAA) to study the anatomical traits based on the paraffin sectioning technique according to Kermanee (2008). The samples were dehydrated in a tertiary butyl alcohol series, infiltrated in liquid paraffin, and embedded in Paraplast. The samples were longitudinally sectioned to 15 µm using a rotary microtome and the sections were stained with a combination of Safranin O and Fast Green. The samples were observed under a light microscope (Olympus, Japan).
Inflorescence Trait Data Collection
Inflorescence samples were wrapped in a mesh bag during development to prevent the loss of flowers. At the time inflorescence had developed to the 80% anthesis stage, the final length and width of the inflorescence samples were recorded using a measuring tape. The number of malformed inflorescences was observed and the percentage of malformation was calculated. The total number of flowers per inflorescence was counted. The proportion of male to hermaphrodite flowers was determined from fully opened flowers and presented as the sex expression ratio. The male and hermaphrodite flowers were randomly measured to determine their flower diameters.
Estimation of Pollen Viability
At the 80% anthesis stage, the hermaphrodite flowers were collected at 8.00–9.00 a.m. Individual flowers were removed from the panicles and stored in FAA; pollen viability was estimated by means of an I2-KI assay according to Kearns and Inouye (1993). The pollen viability was quantified using a light microscope (Olympus, Japan). At least 200 pollen grains per field at 40× magnification were observed with three fields per sample. The numbers of viable pollen grains in each sample were counted and the percentage of pollen viability was calculated.
Estimation of Pollen Germination in the Pistil
The labeled flowers were collected at 11.00–12.00 a.m., approximately 3 h after pollination. Individual flowers were immediately stored in FAA after removal from the panicles. The fixed flowers were washed in de-ionized water and dissected under a stereo-microscope. The pistils were isolated from these flowers and cleared in a 5 M NaOH solution for 1 h at room temperature. Next, the pistils were washed in distilled water and stained with a solution of aniline blue dissolved in 0.1 M K2HPO4 for 10–15 min. The softened pistils were squashed on a slide, after which the fluorescence of the callose was observed under a fluorescence microscope. The total number of flowers that had a pollen tube growing into an ovary was recorded and the percentage of pollen germination in the pistil was calculated.
Determination of Pollen Enzyme Activities
The α-amylase, pectin methylesterase (PME), and Ca2+-ATPase activities were determined to compare differences among the treatments. The anthers were separated from fully opened flowers and the pollen was collected in a Petri dish. The fresh pollen was weighed into 10 mg samples to determine the enzyme activities. α-Amylase activity measurements were determined from reducing sugar using a dinitrosalicylic acid (DNS) assay. The pollen was ground at 0 ̊C with 90 mM NaCl and 30 mM NaHCO3. Then, the homogenate was centrifuged at 5,000×g for 10 min. The supernatants were collected to determine the α-amylase activity (Calzoni and Speranza, 1982). Assay reactions were initiated by adding 1 mL of 1% starch in 20 mM phosphate buffer + 10 mM NaCl (pH 6.9) and 0.05 mL of extracted sample. After 10 min of incubation, an amount of 2 mL of 1% DNS was added, after which the enzymatic reaction was stopped by heating at 100 ̊C for 5 min. The solution was cooled in cold water for 30 min and the extinctions were read at 546 nm (Rick and Stegbauer, 1974). Measurements of PME and Ca2+-ATPase activities were determined in germinated pollens. A 10 mg sample of pollen was cultured in a modified germination medium of the type described in Sukhvibul et al. (1999) which consisted of 200 g·L-1 polyethylene glycol (PEG6000), 0.2 g·L-1 MgSO4, 0.1 g·L-1 KNO3, 0.1 g·L-1 H3BO3, 0.7 g·L-1 Ca(NO3)2, and 150 g·L-1 sucrose. The pollen was cultured for 6 h at 25 ̊C. After 6 h, the germinated pollen was centrifuged at 5,000×g 10 min and the sediment was collected. PME activity was determined using a colorimetric analysis with a pH indicator. The sediment was ground in an ice bath and 100 µL of phosphate buffer (pH 7.5) was added for 30 min. The homogenate was then centrifuged at 5,000×g for 10 min. The supernatants were collected to determine the PME activity. The assay was initiated by adding 2 mL 0.5% of a citrus pectin solution (pH 7.5) and then adding 0.5 mL 0.01% of bromothymol blue in phosphate-buffered saline (pH 7.5) along with 0.83 mL of distilled water and 0.02 mL of the extracted sample. The assay was performed at 25 ̊C for 30 min and the extinctions were read at 620 nm. PME activity was defined as a decrease in absorbance and was expressed as PME activity per gram fresh weight (Hagerman and Austin, 1986; Zocca et al., 2007). For the Ca2+ ATPase activity measurements, the sediment was ground in an ice bath and added to 500 µL 0.9% of NaCl. The Ca2+-ATPase activity was determined following the manufacturer’s instructions with a Ca2+-ATPase assay kit (MyBiosource, Inc., USA).
Estimation of Fruit Set
Inflorescence samples were observed for fruit set. The number of fruit per inflorescence was counted at 7 d after full bloom to avoid any false values from unfertilized fruits.
Statistical Analysis
The experiment was conducted in a complete randomized design, with five replicates for the estimation of inflorescence traits, pollen viability, and pollen germination in the pistil, and three replicates for determination of the enzyme activity. The experimental data of the study were subjected to an analysis of variance (ANOVA). Significant differences were determined using Duncan’s multiple range tests at p < 0.05 with the SPSS 20.0 statistical software (IBM Corp. Released 2011. IBM SPSS Statistics for Windows, Version 20.0. Armonk, NY: IBM Corp).
Results and Discussion
Inflorescence Bud Development
The anatomical study of inflorescence bud development clearly showed rapid growth after the application of EBR and DHECD compared to the control group (Fig. 2). On the second day after the BR application, the EBR application at both concentrations showed more rapid development than the controls. The buds clearly swelled and presented a floral axillary meristem (Fig. 2B and 2C). The 0.01 µM DHECD application produced a swollen bud which was subtended with large floral bracts, and there was also a floral axillary meristem. The bud size was similar to that in the EBR treatments but larger than the controls (Fig. 2D). The 0.1 µM DHECD application resulted in the largest inflorescence bud and the most swollen floral axillary meristem compared to the others (Fig. 2E). On the fourth day after the BR application, the differences in bud development among the treatments were clear. Both EBR treatments showed a swollen floral axillary meristem that was subtended with multiple layers of floral bracts and larger than in the control group (Fig. 2G and 2H). There were no clear differences in developmental outcomes between the EBR concentrations (Fig. 2G and 2H). During this period, the buds treated with DHECD developed most rapidly compared to the control and EBR treatments, as was evident from branching of the inflorescence to become the floral primary and secondary axes (Fig. 2I and 2J). However, no axis meristem was found in either the control cases or after the EBR treatments (Fig. 2F–2H). At the apex of the primary and secondary axes, the emergence of floret primordia was evident in both DHECD treatments (Fig. 2I and 2J). On the eighth day after the application of BR, the floral primary and secondary axes lengthened and inflorescence branches were clearly evident in the BR application treatments. There were more floret primordia after the BR treatments than in the control samples. The floret primordia were swollen and showed evidence of floral organ primordia, specifically sepal, petal and stamen primordia (Fig. 2L–2O). A tertiary axillary meristem appeared in both BR treatments, while no tertiary axillary meristem (nor floral organ primordia) could be found in the control group (Fig. 2K). On the twelfth day after the BR application, the floral organs, in this case sepals, petals, the stamen and the pistil, were present in all treatments. Numerous pollen grains were found in the anthers. At this time, there were no differences in development among the treatments (Fig. 2P–2T). These results revealed that BRs promoted inflorescence development by accelerating floral axillary meristem differentiation and increasing the number of floret primordia. The 0.1 µM DHECD treatment led to the most rapid bud development, especially on the fourth day after the application of BR. It has been reported that BRs promote inflorescence bud development by signaling as a component of axillary meristem formation through BSK1 and BRASSINAZOLE RESISTANT 1 (BZR1). BZR1 is one of the transcription factors that binds to the promoter of the FLOWERING LOCUS D gene and interacts with CYP20-2 proteins to regulate the bud development processes involved in flowering (Zhang et al., 2013). Furthermore, studies have revealed that BRs accelerate flowering by interacting with the gibberellin and abscisic acid pathway signals (Domagalska et al., 2010; Gallego-Bartolomé et al., 2012; Li et al., 2012). In the current study, the rapid growth of the inflorescence bud during initial development was directly influenced by the BRs, consistent with Holá et al. (2010), who reported that exogenous BRs can shorten the time to anthesis in maize when applied at the V6/7 stage.
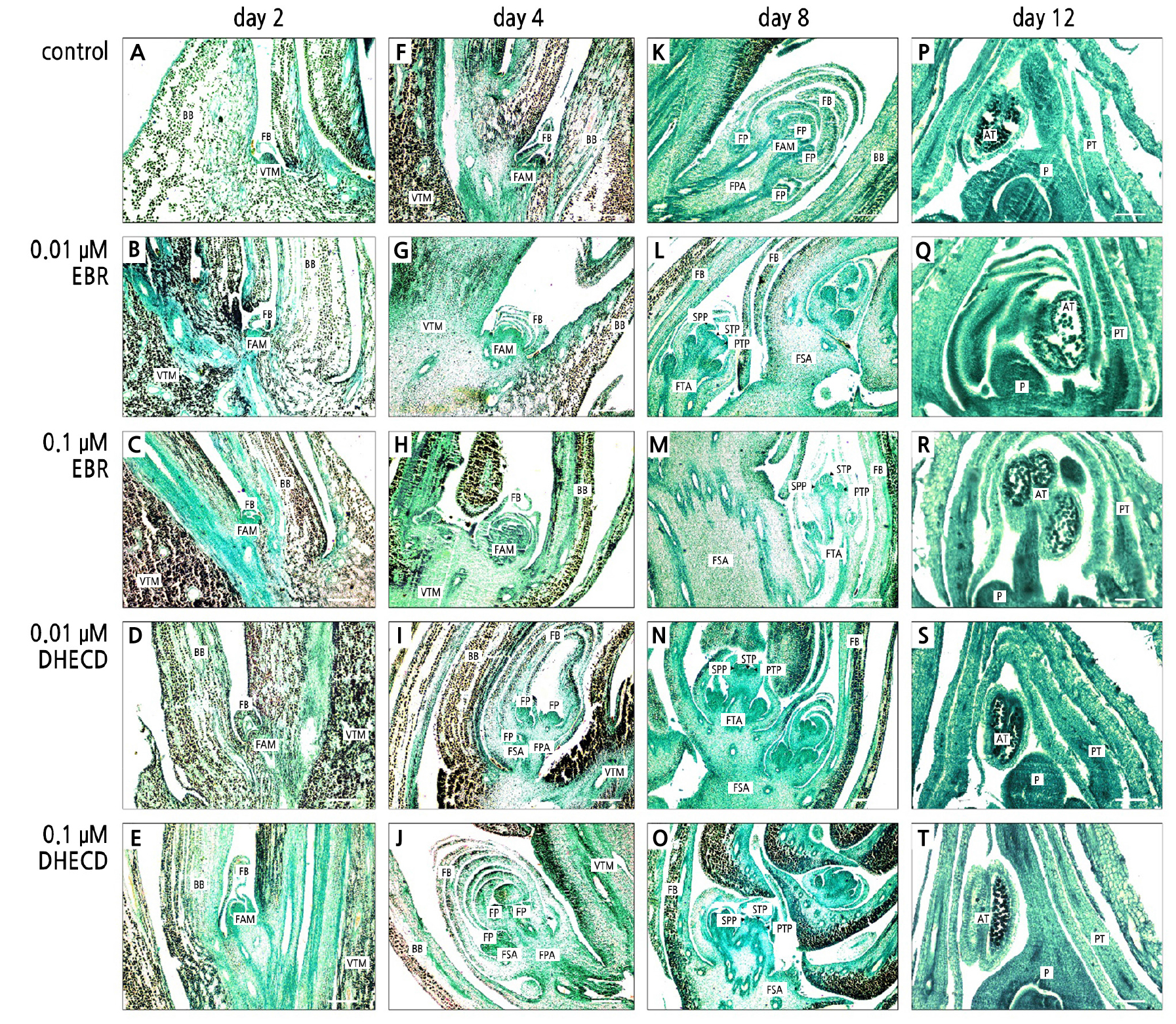
Fig. 2.
Mango inflorescence axillary bud after a treatment with BRs: control on the second day (A); 0.01 µM EBR on the second day (B); 0.1 µM EBR on the second day (C); 0.01 µM DHECD at second day (D); 0.1 µM DHECD on the second day (E); Control on the fourth day (F); 0.01 µM EBR on the fourth day (G); 0.1 µM EBR on the fourth day (H); 0.01 µM DHECD on the fourth day (I); 0.1 µM DHECD on the fourth day (J); Control on the eighth day (K); 0.01 µM EBR on the eighth day (L); 0.1 µM EBR on the eighth day (M); 0.01 µM DHECD on the eighth day (N); 0.1 µM DHECD on the eighth day (O); Control on the twelfth day (P); 0.01 µM EBR on the twelfth day (Q); 0.1 µM EBR on the twelfth day (R); 0.01 µM DHECD on the twelfth day (S) and 0.1 µM DHECD on the twelfth day (T). AT, anther; BB, bud bract; FAM, floral axillary meristem; FB, floral bract; FP, floret primordia; FPA, floral primary axis; FSA, floral secondary axis; FTA, floral tertiary axis; P, pistil; PT, petal; PTP, petal primordia; SPP, sepal primordia; STP, stamen primordia; VTM, vegetative terminal meristem. Scale bars indicate 100 µm.
Inflorescence Traits
The improvement in inflorescence development caused by the BR application directly affected the inflorescence size. The length of the inflorescence was significantly improved by all BR treatments, especially in the 0.1 µM DHECD case (Table 1), as this treatment produced lengths more than 10 cm longer than in the control group (Table 1). EBR and DHECD applications at all concentrations increased the inflorescence width slightly, but this was not significantly different from the controls (Table 1). These results indicated that both BR treatments encouraged the elongation of the primary inflorescence axis but had no clear impact on the secondary axis. BRs improve the inflorescence length by increasing the sensitivity to auxin (Zhang et al., 2009). They act synergistically with auxin to induce cell elongation (Cohen and Meudt, 1983). A higher auxin level is allocated to the primary axis compared to the secondary axis in the shoot (Cieslak et al., 2022). Therefore, the effect of BRs on the primary axis was more pronounced than it was on the secondary axis. BRs reportedly increase cell elongation in many plants, such as in the cucumber (Katsumi, 1985), in soybeans (Clouse et al., 1992), and in groundnut (Vardhini and Rao, 1998). Furthermore, BRs controls the cell elongation and etiolation of Arabidopsis by regulating cytochrome P450 and a dark-induced small G protein (pea Pra2) (Szekeres et al., 1996; Kang et al., 2001). The application of DHECD reportedly increases the length of the Arabidopsis hypocotyl in the dark (Thussagunpanit et al., 2017). Therefore, it appears that DHECD has efficiency similar to EBR with regard to increasing the inflorescence length of mangoes.
The application of DHECD and EBR at all concentrations reduced the occurrence of malformed inflorescences (Table 1). The 0.01 µM EBR and DHECD treatments led to absence of malformed inflorescences. The 0.1 µM EBR and DHECD treatments reduced the percentages of malformed inflorescences from 5% to 2% and 1%, respectively (Table 1). The reduced number of malformed inflorescences stemmed from the stronger development of inflorescence buds, as promoted by the BR application. It has been reported that an application of auxin can reduce the number of malformations in many mango cultivars (Singh, 2000). BRs are known to induce an auxin response in many aspects of plant physiology, such as increased tissue sensitivity to endogenous auxins (Zhang et al., 2009). In the current study DHECD and EBR may have increased the response of the inflorescence bud to self-endogenous auxin, which in turn reduced inflorescence malformation.
Table 1.
Effects of EBR and DHECD, at 0.01 µM and 0.1 µM, on the inflorescence length, inflorescence width, and percentage of malformed inflorescences
Treatment | Inflorescence length (cm) | Inflorescence width (cm) | Malformed inflorescence (%) |
Control | 24.11zcy | 13.71 | 5.00 b |
0.01 µM EBR | 31.74 ab | 15.85 | 0.00 a |
0.1 µM EBR | 29.23 b | 15.59 | 2.00 a |
0.01 µM DHECD | 30.28 b | 15.24 | 0.00 a |
0.1 µM DHECD | 35.40 a | 15.93 | 1.00 a |
Both concentrations of the DHECD treatments provided greater numbers of flowers per inflorescence than in the control group (Table 2). The 0.1 µM DHECD application provided approximately 225% more flowers per inflorescence than the controls (Table 2). However, the total number of flowers per inflorescence after the EBR treatments was not significantly different from that in the control group (Table 2). It appeared that the opportunities for fertilization and fruit set were increased by the DHECD application. According to our anatomical study, DHECD promoted floret primordia formation and axis meristem branching, which were directly related to the high number of flowers produced per inflorescence. Wang et al. (2016) found that BRs modulate cell differentiation in boundary zones that are established at many positions in the plant body, including floral organs. DHECD may affect the boundary zone in ways similar to BRs and may stimulate cell division of the floret primordia, leading to an increased number of flowers in each inflorescence.
BRs had no effect on the sex expression ratio (Table 2). The proportions of male to hermaphrodite flowers were not significantly different among the treatments (Table 2). This result indicated that BRs do not alter sex determination in this mango cultivar, even if applied at the early stage of inflorescence development. This result was consistent with pomegranate flowers, in which 28-homobrassinolide applications did not significantly increase the percentage of bisexual flowers (Gökbayrak and Engin, 2018b). In a pumpkin study, exogenous brassinazole, an inhibitor of brassinosteroid biosynthesis, had no effect on the number of female flowers (Manzano et al., 2011).
The flower diameters did not significantly respond to either BR application in the current study. Both male and hermaphrodite flowers showed flower diameters similar to that of the control group (Table 2). This result was in contrast to that of Nie et al. (2017), who reported that the overexpression of the BR signal receptor SlBRI1 could improve the flower size in tomatoes. BRs promoted petal growth of gerbera by lengthening cells in the middle and basal regions of the petals (Huang et al., 2017).
Table 2.
Effects of EBR and DHECD, at 0.01 µM and 0.1 µM, on the total number of flowers per inflorescence, the flower sex expression ratio, the male flower diameter, and the hermaphrodite flower diameter
Treatment | Total number of flowers | Sex expression ratio | Male flower diameter (mm) | Hermaphrodite flower diameter (mm) |
Control | 597.4zby | 0.257 | 6.64 | 6.80 |
0.01 µM EBR | 1060.2 ab | 0.932 | 6.70 | 6.93 |
0.1 µM EBR | 946.6 ab | 0.177 | 7.13 | 7.10 |
0.01 µM DHECD | 1250.0 a | 0.211 | 6.79 | 7.18 |
0.1 µM DHECD | 1344.2 a | 0.411 | 6.96 | 7.32 |
Pollen Viability
The BRs significantly improved the pollen viability percentage by approximately 10% compared to the controls (Table 3). The 0.1 µM EBR treatment provided the highest percentage of pollen viability (Table 3). From observations under a light microscope, there were more non-viable transparent pollen grains in the controls, followed by the 0.01 µM EBR treatment (Fig. 3A and 3B). Both concentrations of the DHECD treatment and the 0.1 µM EBR treatment produced limited numbers of transparent pollen grains (Fig. 3C and 3E). Pollen viability is an important aspect that is generally used to determine pollen fertility. In the current study, the BR applications increased the pollen fertility of this mango cultivar by improving the pollen viability percentage. This result was consistent with those of various studies in which EBR was used to promote pollen physiological characteristics. For instance, a study of the application of EBR to tomatoes under heat stress concluded that EBR improved pollen viability and helped the pollen become more tolerant to heat (Singh and Shono, 2003). BRs improved the pollen viability of male flowers in a pomegranate study as well (Gökbayrak and Engin, 2018b). Furthermore, EBR and DHECD reduced pollen bursting and increased the percentage of viable pollen in rice under a heat stress condition (Thussagunpanit et al., 2013). The increases in the BR level and BZR1 activity accelerated tapetal degeneration and increased pollen fertility by regulating microspore embryogenesis (Ferrie et al., 2005; Yan et al., 2020). Reduced male fertility and the development of defective pollen resulted from the abnormal tapetum and development of microspores in BR-related mutants (Ye et al., 2010). Numerous studies have determined the genetics and gene expression of pollen involved in viability and germination processes when treated with BRs (Singh and Shono, 2005; Ye et al., 2010; Zhu et al., 2015).
Table 3.
Effects of EBR and DHECD, at 0.01 µM and 0.1 µM, on the percentage of pollen viability, the percentage of pollen germination in the pistil, and fruit set outcomes
Treatment | Pollen viability (%) |
Pollen germination in the pistil (%) |
Fruit set (number of fruits) |
Control | 80.34zdy | 32.00 c | 10.5 b |
0.01 µM EBR | 95.87 b | 53.33 b | 23.1 a |
0.1 µM EBR | 98.68 a | 65.33 ab | 24.4 a |
0.01 µM DHECD | 96.72 ab | 62.67 ab | 18.1 ab |
0.1 µM DHECD | 93.39 c | 70.67 a | 25.4 a |
Pollen Germination in the Pistil
The EBR and DHECD applications improved the pollen germination percentage in the pistil compared to the controls. The application of 0.1 µM DHECD provided the highest percentage of pollen germination in the pistil (70.67%), with this result 38.67% higher than that in the control group (Table 3 and Fig. 4I, 4J). Often, all BR treatments displayed germinated pollen in the pistil with long, straight pollen tubes that could grow into the ovary (Fig. 4C–4J), while the pollen tubes of the control treatment showed less germination development that was insufficient to initiate fertilization in the ovary (Fig. 4A and 4B). These results confirmed that the pollen fertility increased by BR application could promote pollen tube growth into the ovary, consistent with various studies that reported positive effects of BRs on pollen germination, such as in the beautiful-headed carnation (Genç, 2019), grapevines (Gökbayrak and Engin, 2016), and in pomegranates (Gökbayrak and Engin, 2018a).
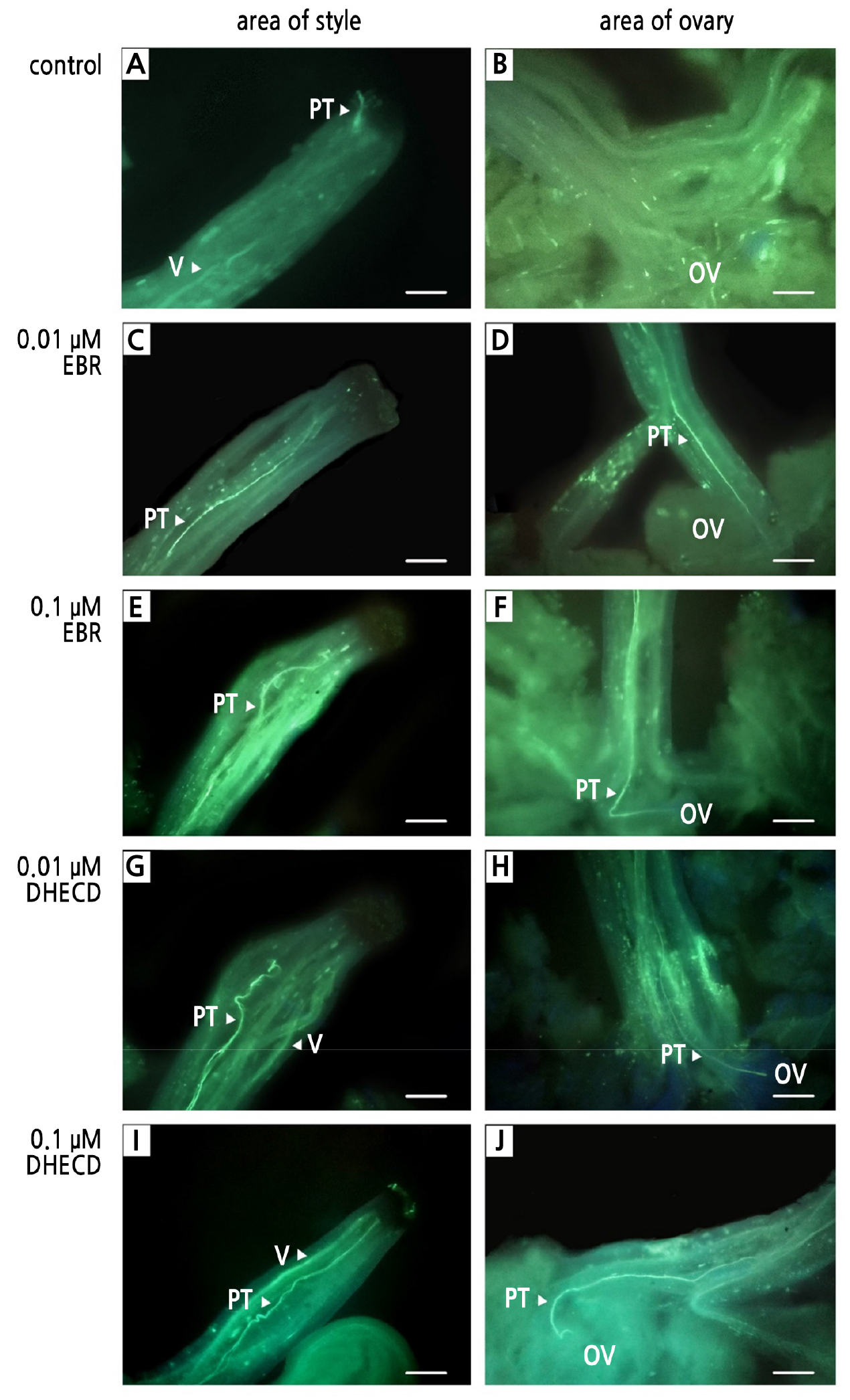
Fig. 4.
Pollen germination and pollen tube growth in the pistil after a treatment with BRs: control at the area of the style (A); control at the area of the ovary (B); 0.01 µM EBR at the area of the style (C); 0.01 µM EBR at the area of the ovary (D); 0.1 µM EBR at the area of the style (E); 0.1 µM EBR at the area of the ovary (F); 0.01 µM DHECD at the area of the style (G); 0.01 µM DHECD at the area of the ovary (H); 0.1 µM DHECD at the area of the style (I) and 0.1 µM DHECD at the area of the ovary (J). PT, pollen tube; V, vascular bundle; OV, ovary. Scale bars indicate 100 µm.
Pollen Enzyme Activities
The α-amylase, PME, and Ca2+-ATPase activities were investigated in the current study. These enzymes play a key role in pollen tube growth. The α-amylase activity is directly related to the starch consumption of pollen when it germinates. It is known that starch is a reserve source of energy for pollen germination. In the current study, there was more α-amylase activity in the 0.1 µM DHECD treatment. However, there were no significant differences in activity with the application of both EBR concentrations and 0.01 µM DHECD compared to the control specimens (Fig. 5A), indicating that pollen treated with 0.1 µM DHECD had more activity to metabolize starch and gain energy to help germination than the other treatments. BRs have been noted to increase germination by enhancing α-amylase activity and starch degradation through the binding of BZR1 and an α-amylase promoter (Hayat et al., 2003; Xiong et al., 2021). PME is one of the key regulators of the stability of apical pollen tube cell walls. PME removes the methyl groups of galacturonic acid polymers and is involved in the plasticity of the cell wall to support polarized tip growth (Bosch et al., 2005). Furthermore, the residue of PME de-esterification can be cross-linked by Ca2+ to rigidify the cell wall structurally (Bosch et al., 2005). In the current study, the application of 0.1 µM DHECD had a stronger positive effect on increasing PME activity levels than the other treatments (Fig. 5B), indicating that DHECD could increase PME activity by playing an important role in promoting pollen tip cell wall formation and finally linking to increased pollen fertility. BRs reportedly increase PME activity in the litchi fruit pericarp (Peng et al., 2004). Ca2+-ATPase is known to play a critical role in the regulation of the Ca2+ gradient between extracellular Ca2+ and intracellular Ca2+ through Ca2+ channels located in the plasma membrane (Franklin-Tong, 1999). The influx and efflux of Ca2+ through these channels are essential for pollen tube growth (Schiøtt et al., 2004; Herbell et al., 2018; Scheible and McCubbin, 2019). Guan et al. (2013) noted that pollen tube elongation was mainly controlled by the tip-specific cytosolic Ca2+ gradient. In the current study, the application of both DHECD concentrations and 0.1 µM EBR improved the activity of Ca2+-ATPase (Fig. 5C), indicating that BR-treated pollen had greater activity in exchanging Ca2+ via the membrane such that more Ca2+ was pumped out of the cell by Ca2+-ATPase activity and deposited into the cell wall. BRs also activate Ca2+ influx by the regulation of Ca2+ signaling (Straltsova et al., 2015). Therefore, the BR treatments increased the efficiency of the growing of the pollen tube compared to the control group. The improvement in Ca2+-ATPase activity following the BR application in the current study was consistent with another study that involved growing peanut seedlings under an iron deficiency condition (Song et al., 2016).
Fruit Set
The application of 0.01 or, 0.1 µM EBR, or 0.1 µM DHECD significantly improved the fruit set percentage compared to the control group. The quantity of fruit produced by these treatments was approximately twice that in the controls (Table 3). This result ensured that there was more successful fertilization resulting from the BRs enhancing pollen enzyme activities, viability and pollen germination in the pistil. The improved fruit set following BR application was consistent with Serna et al. (2012), who reported that BRs increased the yield of pepper by increasing the number of fruits per plant. The foliar application of BRs at the swollen bud stage increased the sweet cherry fruit yield and improved fruit quality (Roghabadi and Pakkish, 2014). Sonjaroon et al. (2018) found that DHECD had effects on plants similar to those of natural BRs. DHECD enhanced rice seed set under heat stress by improving photosynthesis and increasing pollen fertility (Thussagunpanit et al., 2015; Sonjaroon et al., 2018).
Conclusion
Exogenous BRs were effective in enhancing inflorescence development, resulting in a longer length of the panicle and a greater number of flowers per inflorescence. The applications of EBR or DHECD increased pollen enzyme activities, leading to enhanced pollen viability and pollen germination in the pistil. The application of DHECD provided results similar to those of EBR in terms of increases in the inflorescence length, number of flowers per inflorescence, and pollen germination in the pistil, while also reducing the number of malformed inflorescences. Furthermore, compared to EBR, the DHECD application had a stronger beneficial effect on pollen enzyme activities and inflorescence bud development. The 0.1 µM DHECD application was the most effective concentration for enhancing pollen fertility. It was concluded that exogenous EBR and DHECD have potential as an effective solution to deal with the low fruit set problem in mangoes. Further studies are needed to evaluate the efficiency of EBR and DHECD in promoting fruit development and reducing fruit drops in various fruit trees, which would result in improved yields.