Introduction
Materials and Methods
Experimental environment and materials
Growth characteristics
Photosynthetic efficiency analysis
Chlorophyll content analysis
Chlorophyll fluorescence response analysis
Microscopic analysis of the leaf surface structure
Quantitative analysis of pharmacological components
Statistical analysis
Results and Discussion
Growth characteristics
Physiological response characteristics
Leaf surface microstructure
Pharmacological characteristics
Conclusion
Introduction
Climate change is a pressing global concern that significantly influences plant ecosystems and physiology. Its overarching effects can potentially modify physiological growth mechanisms, growth trajectories, biomass yields, chemical compositions, and the quality and safety of medicinal plants (Patni et al., 2022). Most notably, climate change can influence the rate of development in key phenological stages through temperature fluctuations and can directly damage plant tissues (Hatfield and Prueger, 2015; Vetharaniam et al., 2022). Temperature is a crucial climatic factor that significantly influences plant growth and development, including germination, leafing, flowering, and maturity, thereby affecting productivity. Temperature changes not only affect the quantitative and qualitative value of plants (Patni et al., 2022) but can also induce various stresses on the growth, development, final yields, and pharmacological functions of medicinal plants (Shahrajabian et al., 2023). Extremely high and low temperatures typically induce physiological and functional impairments, leading to diminished yields and compromised quality levels (Liu et al., 2019; Ren et al., 2019).
Astragalus membranaceus Bunge, commonly known as Hwang-gi in Korea, Japan, Russia, Taiwan, Mongolia, and Central Asia, is an essential herb for promoting health (Zhang et al., 2011; Li et al., 2017; Jeong et al., 2020). The roots of Hwang-gi have a significant historical legacy, and Hwang-gi has been recognized as a health-promoting herb for more than 2000 years (Shao et al., 2004; Fu et al., 2014). Especially recognized on a global scale, Hwang-gi roots stand as a vital reservoir of medicinal constituents and supplements for dietary use (Zhang et al., 2011; Li et al., 2017; Yu et al., 2019). Recent studies have isolated essential physiologically active compounds from Hwang-gi roots, such as triterpene saponins (astragalosides), isoflavonoids (formononetin and calycosin), and polysaccharides, which demonstrate significant physiological activity through antioxidant mechanisms to prevent tissue damage (Zhang et al., 2011; Fu et al., 2014; Shahzad et al., 2016; Jeong et al., 2020). Notably, astragaloside IV (Gai et al., 2017; Wang et al., 2019; Wu et al., 2020; Liang et al., 2023) and calycosin-7-O-glucoside (Pan et al., 2015; Gai et al., 2019) are prominent constituents exhibiting a broad spectrum of pharmacological properties, including immune enhancement, antioxidant, anti-inflammatory, and anti-diabetic effects while also demonstrating the ability to modulate various signaling pathways (Xiao et al., 2011; Zhang et al., 2014; Yang et al., 2019; Wu et al., 2020; Sun et al., 2021).
However, recent climate change has adversely affected Hwang-gi cultivation owing to sudden increases in high-energy UV-B radiation, daily maximum temperatures, and extreme climatic events (Kim et al., 2020b; Hatfield and Prueger, 2015; Patni et al., 2022; Pandey et al., 2022). Rising temperatures can cause shifts in the primary cultivation areas of major crops, whereas droughts and heavy rainfall can negatively affect Hwang-gi production. Furthermore, the lack of standardized growth conditions for commercial Hwang-gi cultivation may affect its quality and functional properties (Ren et al., 2019). Temperature influences various aspects of plants, including the length, diameter, growth, yield, biomass, and quality. Plant metabolic pathways are the major targets of temperature stress (Ren et al., 2019). High temperatures induce heat stress, impair photosynthetic capacity, limit plant growth, and alter biochemical and molecular processes, thereby altering the concentrations of secondary metabolites in plants (Zobayed et al., 2005). Consequently, the quality and functional properties of Hwang-gi are closely related to ecological factors such as climate and soil conditions (Yang et al., 2020). Given the threat to Hwang-gi production in key domestic regions cultivating medicinal plants due to climate change, this study aimed to systematically analyze the physiological and pharmacological changes in Hwang-gi in response to temperature variations. Specifically, conducted within a temperature-gradient greenhouse, this study seeks to evaluate Hwang-gi’s resilience and adaptability to rising temperatures. The primary objective of this study was to determine the effects of temperature on the medicinal value and safety of Hwang-gi. By comprehensively understanding the physiological and pharmacological responses of Hwang-gi to climate change, fundamental data can be provided to support stable Hwang-gi production under future climatic conditions.
Materials and Methods
Experimental environment and materials
This study was conducted from the cultivation of seedlings on May 14, 2021 to harvesting on August 27, 2021 at the National Institute of Horticultural and Herbal Science, Ginseng & Medicinal Crops Research Institute’s test plot located in Eumseong, Chungcheongbuk-do, utilizing a semi-closed complex environmental control system known as a temperature-gradient greenhouse (dimensions: width 2.6 m × height 2.5 m × length 27 m) (Kim et al., 2020b). The temperature-gradient greenhouse serves as a simulated facility for a warm climate, capable of creating a 0 to 5°C difference in the ambient temperature within the same enclosed space using air and exhaust fans (Batts et al., 1998; Salazar-Parra et al., 2015). By examining the photosynthetic characteristics, growth features, and pharmacological properties of Hwang-gi, a representative medicinal plant in South Korea, this study aimed to investigate the effects of increasing temperatures on the overall growth of Hwang-gi in response to climate change and to explore methods to minimize the influence of temperature.
The soil within the temperature-gradient greenhouse was plowed to a depth of 20 cm. Hwang-gi was planted in double rows at a height of 20 cm and width of 57 cm at intervals of 30 cm in the morning and at 15 cm during the day. Growth was maintained for four months, with a total of four temperature zones arranged in 30 repetitions per temperature zone. Fertilizers (2,000 kg of compost, 17 kg of nitrogen, 11 kg of phosphorus, and 14 kg of potassium) were applied before planting Hwang-gi seedlings and were thoroughly mixed into the soil. Soil moisture was uniformly maintained through localized irrigation every three to four days during the growth period. The temperature and solar radiation level were measured using temperature and light sensors installed 2 m above the ground, with data loggers storing information at hourly intervals. Additionally, the time–domain reflectometry (TDR) method, a technique for measuring electrical characteristics, was employed to monitor changes in the soil moisture content and electrical conductivity, utilizing the ideal soil sensor TRIME-PICO64 (IMKO Micro GmbH, Germany) across different temperature zones.
The temperature difference between the front and rear of the temperature-gradient greenhouse was maintained at 5.1 to 5.3°C higher than the ambient temperature at the entrance. One-month-old Hwang-gi seedlings were zigzag-planted from the front to the rear. The temperature zones were set at every quarter point from the front to the rear of the temperature–gradient greenhouse, labeled as T1 (ambient temperature + 0–1.8°C, the control group), T2 (ambient temperature + 2.3–2.5°C), T3 (ambient temperature + 3.3–3.5°C), and T4 (ambient temperature + 5.1–5.3°C) (refer to Table 1 and Fig. 1). The average, maximum, and minimum temperatures during the experimental period are listed in Table 1. The soil temperatures at midday were respectively 3.34°C, 4.3°C, and 6.3°C higher in T2, T3, and T4 compared to the control group, T1 (Table 1).
Table 1.
Daily temperature variations during the growing season
Test site | Temperature | May | June | July | August | Tmean |
Tair | Tavg (°C) | 15.74 | 21.77 | 25.94 | 24.45 | 21.98 |
T1 | Tavg (°C) | 17.37 | 23.53 | 27.68 | 26.22 | 23.70 |
Tmax (°C) | 24.25 | 30.23 | 34.31 | 32.06 | 30.21 | |
Tmin (°C) | 11.51 | 18.41 | 22.93 | 22.20 | 18.76 | |
T2 | Tavg (°C) | 18.27 | 24.27 | 28.25 | 26.9 | 24.42 |
Tmax (°C) | 24.53 | 30.17 | 34.00 | 32.25 | 30.24 | |
Tmin (°C) | 12.66 | 19.49 | 24.07 | 23.27 | 19.87 | |
T3 | Tavg (°C) | 19.23 | 25.17 | 29.23 | 27.95 | 25.40 |
Tmax (°C) | 25.74 | 31.38 | 35.25 | 33.42 | 31.45 | |
Tmin (°C) | 13.43 | 20.30 | 24.85 | 24.25 | 20.71 | |
T4 | Tavg (°C) | 20.90 | 27.01 | 31.22 | 29.56 | 25.88 |
Tmax (°C) | 27.98 | 34.18 | 38.46 | 35.46 | 34.02 | |
Tmin (°C) | 14.69 | 21.63 | 26.19 | 25.51 | 22.01 |
Tavg, Tmax, and Tmin represent the monthly average, maximum, and minimum temperatures, respectively, during the experimental periods; Tmean represents the mean temperature of the growing season (May to August). Note: T1, increase by about 0‒1.8°C from ambient temperatures; T2, increase by about 2.3‒2.5°C from ambient temperatures; T3, increase by about 3.3‒3.5°C from ambient temperatures; and T4, increase by about 5.1‒5.3°C from ambient temperatures.
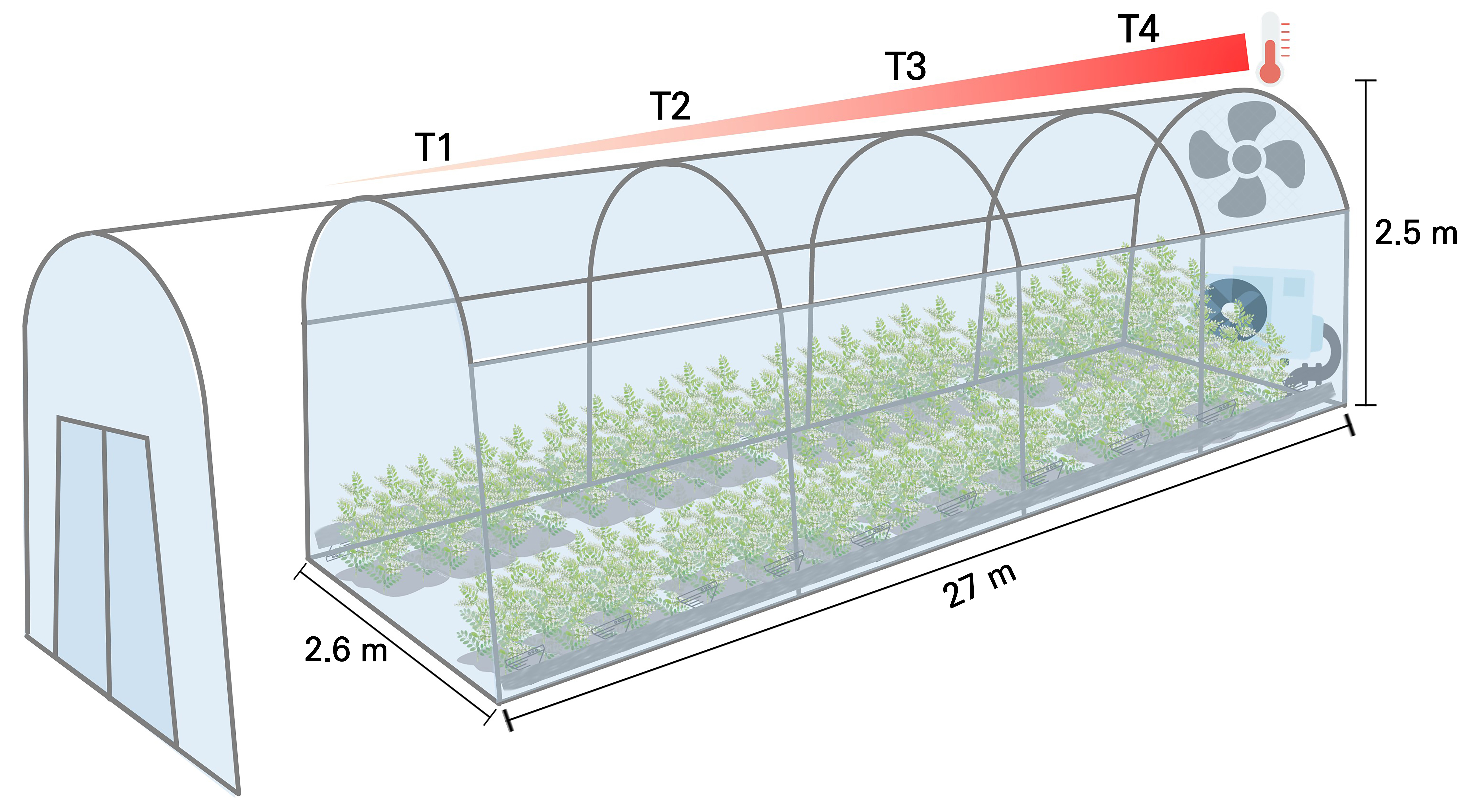
Fig. 1.
Overview of the temperature-gradient greenhouse equipped with a heating actuation system. Note: T1, increase by about 0‒1.8°C from ambient temperatures; T2, increase by about 2.3‒2.5°C from ambient temperatures; T3, increase by about 3.3‒3.5°C from ambient temperatures; and T4, increase by about 5.1‒5.3°C from ambient temperatures.
Growth characteristics
The growth responses of Hwang-gi to the temperature treatments were assessed by selecting five individual specimens and measuring their shoot length and fresh and dry weights of the aerial and underground parts. At the tenth week of the temperature treatment, the shoot length was measured using a folding ruler (2 m) while the fresh and dry weights were measured on the initiation and termination of the temperature treatment. After harvesting, the fresh weights of the aerial and underground parts were measured separately using an electronic scale. Subsequently, the dry weights were determined by drying the plant material at 75°C in a drying oven for 72 hours and measuring the weight afterward. The relative growth rate (RGR) was calculated for the dry weights of the aerial and underground parts of the five individual specimens from the initiation of planting to the termination of the 105th day using the following formula:
RGR, g·g-1·d-1 = (LnW2 – LnW1) / (t2 – t1),
where W2 denotes the dry weights of the aerial and underground parts at the termination of the experiment, W1 represents the dry weights of the aerial and underground parts at the initiation of the experiment, and t1 and t2 are correspondingly the initiation and termination dates of the experiment.
Photosynthetic efficiency analysis
The physiological parameters of the leaves, in this case the net photosynthetic rate (Pn, μmol·CO2·m-2·s-1), stomatal conductance (Gs, mol·H2O·m-2·s-1), and transpiration rate (Tr, mmol·H2O·m-2·s-1), were measured using a LI-6400XT portable photosynthesis system (LI-6800XT, Li-Cor, Lincoln, NE, USA) at ten weeks after the treatment between 09:00 and 12:00, when the stomata were not influenced. All measurements were conducted on the second to fourth fully expanded leaves from the apical buds of five individual specimens per temperature treatment in a controlled-environment chamber. The instantaneous water use efficiency (WUE, mmol·mol-1) was defined as the Pn/Tr ratio (Sinclair et al., 1984). During the gas exchange measurements, the photosynthetic photon flux density (PPFD) was maintained at 1000 μmol·m-2·s-1, the chamber temperature was 27°C, the relative humidity was 50–60%, and the CO2 concentration was held at 400 μmol·mol-1 using a CO2 injector system.
Chlorophyll content analysis
The chlorophyll contents of Hwang-gi across the different temperature treatments were measured as the leaf chlorophyll concentration in µmol per m² of leaf area, focusing on leaves for which photosynthesis measurements were completed. The measurements were conducted using the non-destructive chlorophyll concentration measurement tool MC-100 (Apogee Instruments, Inc., Logan, UT, USA) on intact leaf samples, allowing accurate measurements of chlorophyll concentrations without causing damage to the leaves.
Chlorophyll fluorescence response analysis
The chlorophyll fluorescence responses of Hwang-gi leaves across the different temperature treatments were measured using a chlorophyll fluorometer (Pocket PEA, PEA plus V1.10, Hansatech Instrument Ltd., Norfolk, UK) on fully expanded leaves selected at the tenth week post-treatment between 10:00 and 11:00 AM. Prior to the fluorescence measurements, a leaf clip was attached to the leaves for a 30-minute dark adaptation period. Following dark adaptation, a saturated pulse of 3,500 µmol-2·s-1 was applied to the upper leaf surface to measure the performance index considering the absorption basis (PIABS). PIABS is a photosynthetic parameter that reflects the efficiency of energy conversion during the reduction of photosystem II (PSII) and photosystem I (PSI) electron acceptors by absorbed photons (Kwak et al., 2018).
Microscopic analysis of the leaf surface structure
The microstructural characteristics of the Hwang-gi leaves were examined using a field-emission scanning electron microscope (FESEM). Leaf samples were collected from the fully expanded leaves of Hwang-gi grown in a temperature-gradient greenhouse at the National Institute of Horticultural and Herbal Science of the Rural Development Administration in Eumseong. Five fully expanded leaves were collected from each temperature treatment, placed in a Petri dish, and transferred to an icebox. After transportation to the research laboratory at the University of Seoul, the leaf samples were pretreated for surface observation. Leaf sections consisting of adaxial and abaxial surfaces with a midrib and secondary veins were cut into 5 mm × 20 mm pieces and attached to conductive double-sided carbon tape on an electron microscopy stub. To preserve the morphological features of the leaf surface, the samples were dehydrated using a freeze dryer (FD-8508, Ilshinbiobase, Co., Ltd., Korea) for 24 h. The stubs with the dehydrated leaf sections were stored in a desiccator chamber at controlled temperature and humidity levels until the observation step, at which time the samples were coated with platinum using an ion sputter coater (Ion-sputter, MC1000, Hitachi, Japan) and observed under a scanning electron microscope (SU8010, Hitachi High-Tech, Tokyo, Japan) at three magnifications (×60, ×300, ×2000). After imaging, a 3D surface analysis optical microscope (Bruker, Contour GT-K) was used to measure the leaf surface roughness for inter-species comparisons.
Quantitative analysis of pharmacological components
In our study, marker compounds in underground part (root) of Hwang-gi were analyzed at the Natural Product Institute of Science and Technology, which has the technology to separate and purify highly pure indicator substances from natural products. A detailed description of the analytical procedure is described below. The roots of Hwang-gi were weighed after different temperature treatments and subjected to three rounds of reflux cooling extraction using 100% ethanol at a 20-fold volume. Subsequently, 60 mg of the root extracts from each treatment were dissolved in 100% methanol to prepare a 15 mg/mL solution (15000 ppm). Individual standard compounds (Fig. 2), specifically methylnissolin-3-O-β-d-glucopyranoside (CAS No. 94367-42-7), calycosin-7-O-glucoside (CAS No. 20633-67-4), and formononetin (CAS No. 485-72-3), were precisely weighed at 1 mg each and dissolved in pure methanol to prepare a 0.25 mg/mL standard solution (250 ppm). Separately, another set of standard compounds, in this case calycosin (CAS No. 20575-57-9), astragaloside I (CAS No. 84680-75-1), and astragaloside IV (CAS No. 84687-43-4), was accurately weighed at 1 mg in each case and dissolved in pure methanol to prepare a 0.5 mg/mL standard solution (500 ppm). The standard solutions were diluted with 100% methanol. Root samples were extracted using a Waters e2695 Alliance HPLC system (Waters, Milford, MA, USA) equipped with an evaporative light scattering detector (HPLC-ELSD) at a temperature of 35°C. Specifically, the analysis was conducted in a YMC Pack-Pro C18 column (4.6 mm × 250 mm, 5 μm, Agilent, CA, United States). The injection volume of the sample was 10 µl. A mixture of solvent A (0.1% formic acid in water) and solvent B (acetonitrile) was used as a mobile phase. The optimized gradient elution was as follows: 0–10 min 80% A, 20% B; 10–15 min 60% A, 40% B; 15–20 min 55% A, 45% B; 20–30 min 50% A, 50% B; 30–35 min 45% A, 55% B; 35–38 min 0% A, 100% B; 38–43 min 0% A, 100% B; 43–45 min 80% A, 20% B; and 45–60 min 80% A, 20% B.
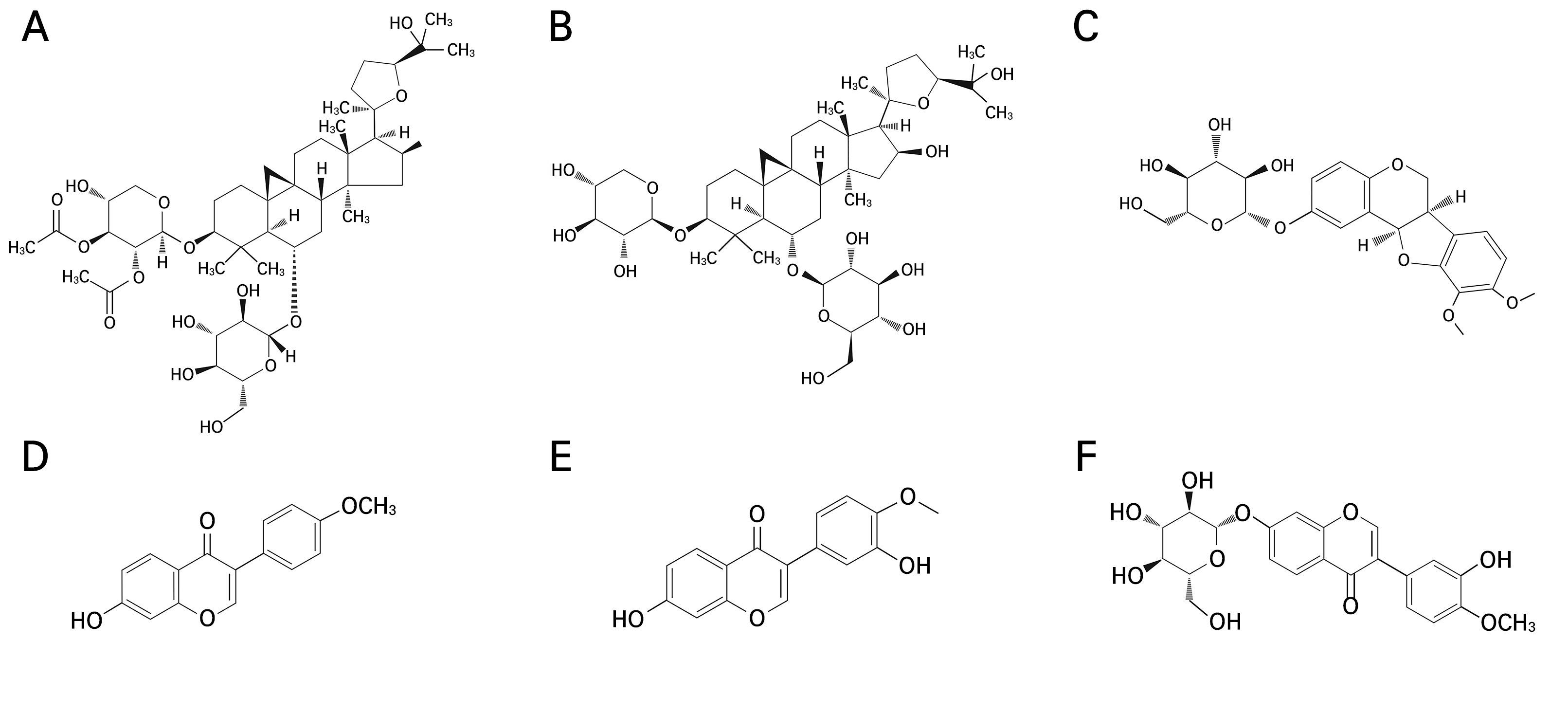
Fig. 2.
Chemical structures of the major compounds identified from Astragalus membranaceus roots (Zheng et al., 2015; Salehi et al., 2021; Wu et al., 2021; Zhang et al., 2022). (A) astragaloside I (Molecular Formula: C45H72O16, CAS No. 84680-75-1); (B) astragaloside IV (Molecular Formula: C41H68O14, CAS No. 84687-43-4); (C) methylnissolin-3-O-β-d- glucopyranoside (Molecular Formula: C23H26O10, CAS No. 94367-42-7); (D) formononetin (Molecular Formula: C16H12O4, CAS No. 485-72-3); (E) calycosin (Molecular Formula: C16H12O5, CAS No. 20575-57-9); and (F) calycosin-7-O-glucoside (Molecular Formula: C22H22O10, CAS No. 20633-67-4).
Statistical analysis
All data were analyzed using IBM SPSS Statistics 28 (IBM Corp., Chicago, IL, USA). A one-way analysis of variance (ANOVA) was conducted to determine significant differences between the groups. Tukey’s HSD test (p < 0.05) was used to assess the significance of the mean differences. The results are expressed as mean ± standard deviation (SD).
Results and Discussion
Growth characteristics
Climate change affects the biochemical composition of plants throughout their life cycle, including their growth and maturation stages. Temperature is a major environmental factor that affects plant physiological processes, particularly photosynthesis and growth (Zobayed et al., 2005). Fig. 3 illustrates the soil temperature and conductivity at 15 and 40 days after transplantation in August of 2018. The soil moisture content decreased in the following order over time: T1, p < 0.001; T2, p < 0.001; T3, p < 0.001; and T4, p < 0.001 (Fig. 3A). The variations in soil moisture across the temperature intervals exhibited distinct values contingent on the assessed time frames. However, as a general trend, the soil moisture content of T1 was consistently higher than those of the other temperature treatments (T2, T3, and T4), as illustrated in Fig. 3A. In more detail, the range of soil moisture from the temperature gradients decreased by 32.8% for T2, 35.5% for T3, and 31.6% for T4 as compared to that of T1 after 15 days of temperature exposure, while after 40 days of temperature exposure, the corresponding relative values were reduced by 22.6%, 43.1%, and 25.0%. Soil conductivity did not show statistically significant differences between the treatments after 15 days of temperature exposure, but after 40 days of temperature exposure, T2, T3, and T4 showed significantly lower values than T1 (Fig. 3B).
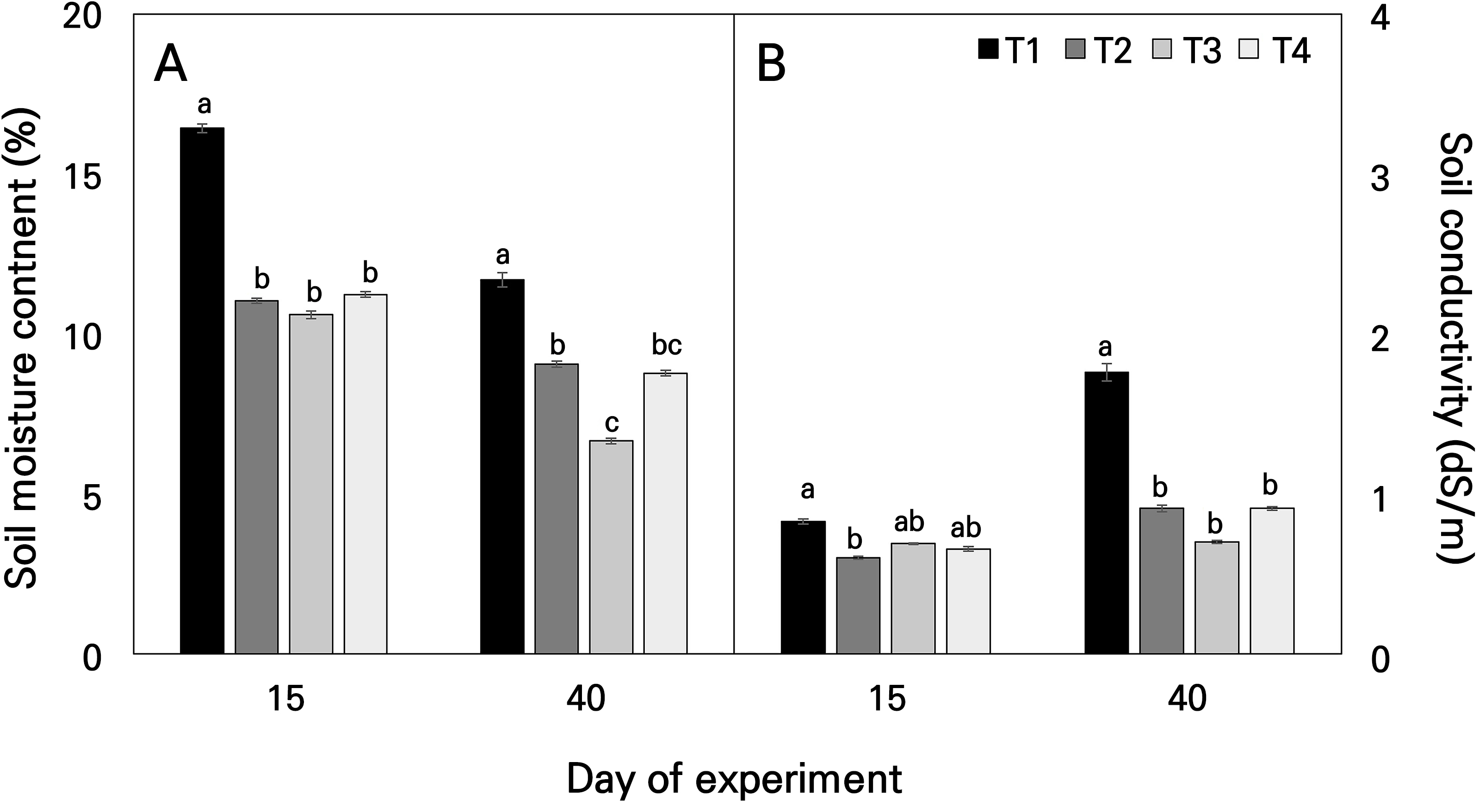
Fig. 3.
Average soil water content (A) and soil conductivity (B) during the growth stage for Astragalus membranaceus monitored by a portable soil moisture sensor under different temperature conditions. Data represent the means ± SE of four replicate plants. Different letters on the bars denote significant differences at the level of 0.05. T1, T2, T3, and T4 represent each temperature section in the temperature-gradient greenhouse depicted in Figure 1.
The relative growth rates (RGRs) by temperature interval showed that growth decreased as the temperature was increased, as depicted in Figs. 4 and 7. After harvesting the Hwang-gi, the fresh weight and dry weight of the plant organs were measured. The fresh weight of T1 was highest at 284.2 g for the aerial parts and 43.2 g for the underground parts, and the dry weight was highest at 91.5 g for the aerial parts and 12.1 g for the underground parts. The root fresh weights of T3 and T4 decreased to 63% and 75% of those of T1, respectively, whereas the corresponding root dry weights decreased by 58% and 75%. In addition, the fresh weights of the aerial parts in T3 and T4 decreased to 66% and 68% of that of T1, while the corresponding dry weights of aerial parts in T3 and T4 decreased to 58% and 57%. These results confirmed a significant decrease in both the fresh and dry weights in the temperature-elevated treatment groups (Figs. 4 and 7).
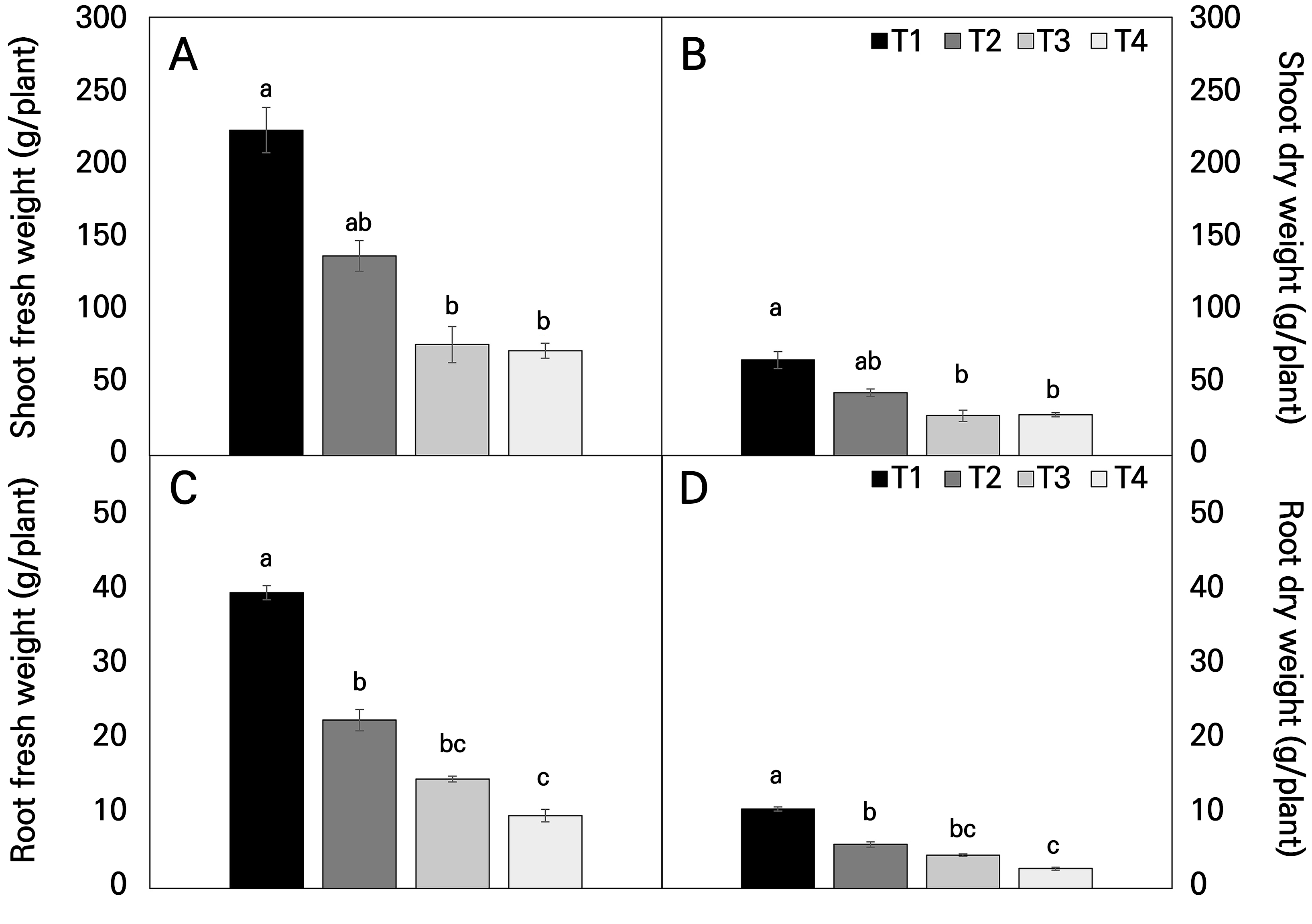
Fig. 4.
Shoot fresh weight (A), shoot dry weight (B), root fresh weight (C), and root dry weight (D) of Astragalus membranaceus at harvest under different temperature conditions. Data represent the means ± SE of four replicate plants. Different letters on the bars denote significant differences at the level of 0.05. T1, T2, T3, and T4 represent each temperature section in the temperature-gradient greenhouse depicted in Figure 1.
Growing degree days (GDD) is a widely used indicator for estimating crop growth during the growing season and for determining plant phenological events such as flowering, maturity, and harvest dates (McMaster and Wilhelm, 1997). Agroclimatologists also use the effective accumulated temperature (EAT) to express the heat needed for plant development stages. EAT features prominently in models predicting individual plant development stages, a crucial component of crop management. As expected, both GDD and EAT increased in all temperature gradient house treatments from May to June (Table 2). Our data suggest that rising atmospheric temperatures will limit soil moisture and create conditions for heat stress. While high temperatures (T4) may initially accelerate Astragalus germination and basal growth in future environments, the mid-term growth stage could experience simultaneous soil moisture and heat stress due to high air temperatures and soil drying, particularly at higher treatment levels.
Table 2.
Variability of growing degree days as a climate impact indicator under temperature gradient tunnel environments
Stage/Variable | T1 | T2 | T3 | T4 |
May | 163.5 | 179.6 | 196.0 | 225.3 |
June | 406.0 | 428.1 | 455.0 | 510.3 |
July | 548.0 | 565.6 | 596.2 | 657.8 |
August | 450.0 | 467.9 | 496.7 | 540.5 |
EAT (°C) z | 1,567.5 | 1,641.2 | 1,743.8 | 1,934.0 |
GDD (day) y | 1,782 | 1,852 | 1,978 | 2,216 |
z EAT, effective accumulated temperature (°C); y GDD, growing degree days (day). Note: T1, increase by about 0–1.8°C from ambient temperatures; T2, increase by about 2.3–2.5°C from ambient temperatures; T3, increase by about 3.3–3.5°C from ambient temperatures; and T4, increase by about 5.1–5.3°C from ambient temperatures.
Drought stress, a major limiting factor for plant productivity arising from a combination of water scarcity and high temperatures (Kyratzis et al., 2017), is expected to increase uncertainty as regards crop yields owing to future climate change. Moreover, global warming due to CO2 can drastically alter extreme climate responses, potentially amplifying drought risks and increasing heatwaves and wildfires (Kim et al., 2020a). Low soil moisture, in conjunction with rising atmospheric temperatures, tends to limit the latent heat flux and accelerate surface drying, as observed in this study. Such limitations in soil moisture owing to temperature increases can affect plant physiological processes, altering the production and accumulation of secondary metabolites (Garg et al., 2001; Khan et al., 2010). When plants undergo water scarcity, they maintain photosynthesis and cell turgor for a short time; however, prolonged water scarcity can lead to drought stress, resulting in stomatal closure, a reduced leaf size, and the inhibition of plant growth (Boyer, 1970; Farooq et al., 2012; Lin et al., 2022).
Physiological response characteristics
Fig. 5A shows the changes in the main photosynthetic pigment values of Hwang-gi leaves grown under different temperature gradients in the temperature-gradient greenhouse. An examination of the variation in the chlorophyll content in Hwang-gi leaves revealed a decrease in the chlorophyll content with an increase in the temperature (Fig. 5A). The total chlorophyll content differed across the temperature treatment zones, where T2 exhibited a chlorophyll content similar to that of T1, with T3 and T4 showing significant decreases of 20% and 26%, respectively, compared to T1.
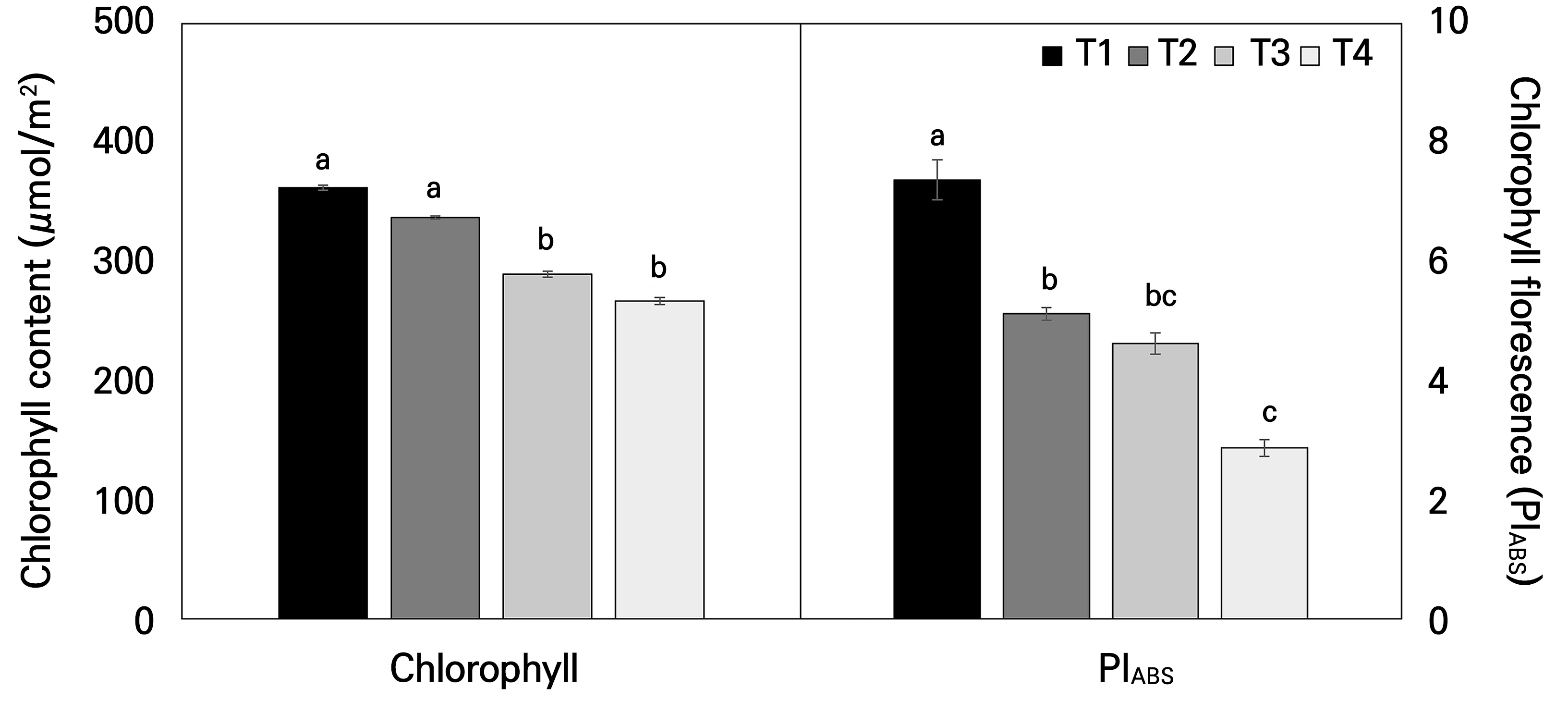
Fig. 5.
Chlorophyll content (A) and chlorophyll fluorescence (B) of Astragalus membranaceus leaves of plants exposed to different temperature conditions for 21 days. Data represent the means ± SE of 25 replicate plants. Different letters on the bars denote significant differences (p < 0.05). T1, T2, T3, and T4 represent each temperature section in the temperature-gradient greenhouse depicted in Figure 1.
The chlorophyll fluorescence parameter, PIABS, which represents the photochemical performance index on an absorption basis, showed a significant decrease in the elevated temperature ranges of T3 and T4 compared to the control group T1 (Fig. 5B). T2 showed a 30.5% decrease compared to T1, while T3 saw a 37.2% reduction. T4, however, displayed a more pronounced decline of 61.1%. Chlorophyll fluorescence, a swift non-destructive tool for evaluating PSII stress responses (Mathur et al., 2011), reveals the photochemical and heat dissipation efficiency of plants (Maxwell and Johnson, 2000). Importantly, PIABS, which is a measure of the three independent functional stages of photosynthesis, energy absorption (reaction center density of the chlorophyll layer), excitation energy trapping, and the conversion of excitation energy to the electron transport step, is a parameter very sensitive to a variety of environmental stress conditions in plants (Strasser, 1997; Tsimilli-Michael et al., 2000; Oukarroum et al., 2007; Kalaji et al., 2017). Hence, PIABS, as a comprehensive indicator of photosynthetic performance, is known to decrease with an increase in the temperature, further confirming leaf damage caused by the elevated temperatures in this study.
Temperature stress can have a significant effect on plant physiological responses, especially when leaves are exposed to high temperatures. This can lead to protein denaturation, membrane destruction, electron transport disruption, and increased reactive oxygen species (ROS), all of which contribute to reduced photosynthetic efficiency over time (Kalaji et al., 2017). High-temperature stress can denature proteins and disrupt cell membranes, thereby impairing the functionality of photosynthetic enzymes. Moreover, heat stress caused by high temperatures may lead to excessive ROS accumulation, which can damage the photosynthetic apparatus. Exposure to high temperatures can trigger non-photochemical chlorophyll fluorescence quenching (NPQ), a process in which excessive light energy is converted into heat, causing a reduction in PIABS. NPQ dissipates excess thermal energy, diminishing the available light energy for photosynthesis and ultimately reducing PIABS and adversely affecting the photosynthetic capabilities of plants.
In the present study, gas exchange measurements of the Hwang-gi plants grown in different temperature ranges in the temperature-gradient greenhouse showed a clear difference in the photosynthetic capacity with an increase in the temperature (Fig. 6). The photosynthetic rate (Pn), transpiration rate (Tr), and water use efficiency (WUE) in T1 closely resembled those observed in T2. Nevertheless, the stomatal conductance (Gs) of T2 exhibited a significant increase compared to that of T1. However, except for Gs (Fig. 6B), the observed variations were not statistically significant, implying that the photosynthetic capacity in the T2 treatment closely resembled that in the T1 treatment. Importantly, the T4 treatment, with the highest temperature increase, showed significant decreases in Pn (p < 0.001), Gs (p < 0.001), Tr (p = 0.001), and WUE (p < 0.001) compared to T1. Climate change and global warming are expected to increase temperatures, exacerbate heat stress in plants, and limit productivity and biomass production. Of all cellular processes in plants, photosynthesis is the most vulnerable to temperature increases (Sharkey and Schrader, 2006). Additionally, these high temperatures alter the oxidation-reduction characteristics of PSII reaction centers, reducing the efficiency of photosynthetic electron transport in both photosystems (Mathur et al., 2014) and exacerbating the challenges faced by plants.
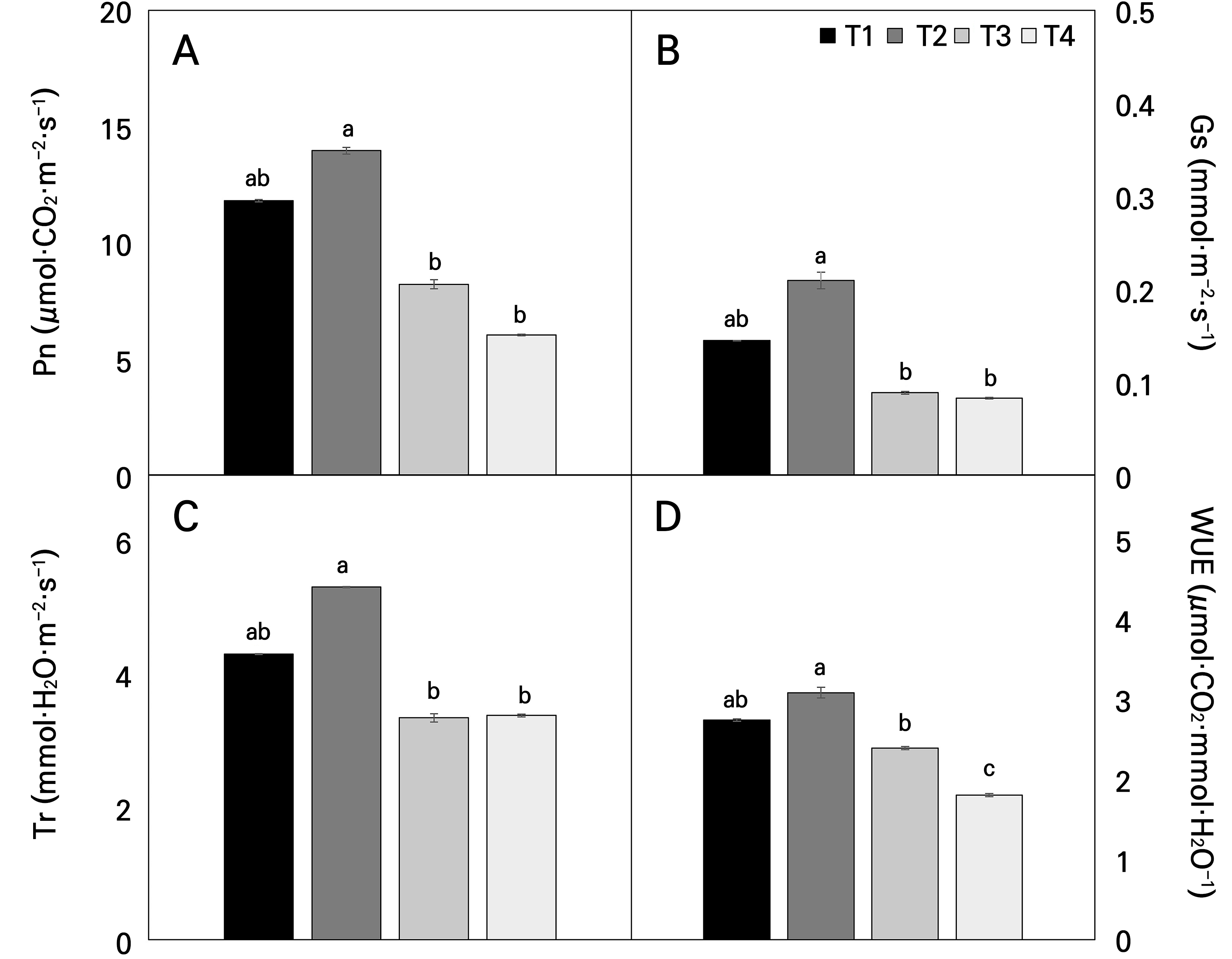
Fig. 6.
Photosynthetic parameters of the net photosynthesis (Pn) (A), stomatal conductance (Gs) (B), transpiration rate (Tr) (C), and water use efficiency (WUE) (D) of Astragalus membranaceus leaves exposed to different temperature conditions for 21 days. Data represent the means ± SE of 25 replicate plants. Different letters on the bars denote significant differences (p < 0.05). T1, T2, T3, and T4 represent each temperature section in the temperature-gradient greenhouse depicted in Figure 1.
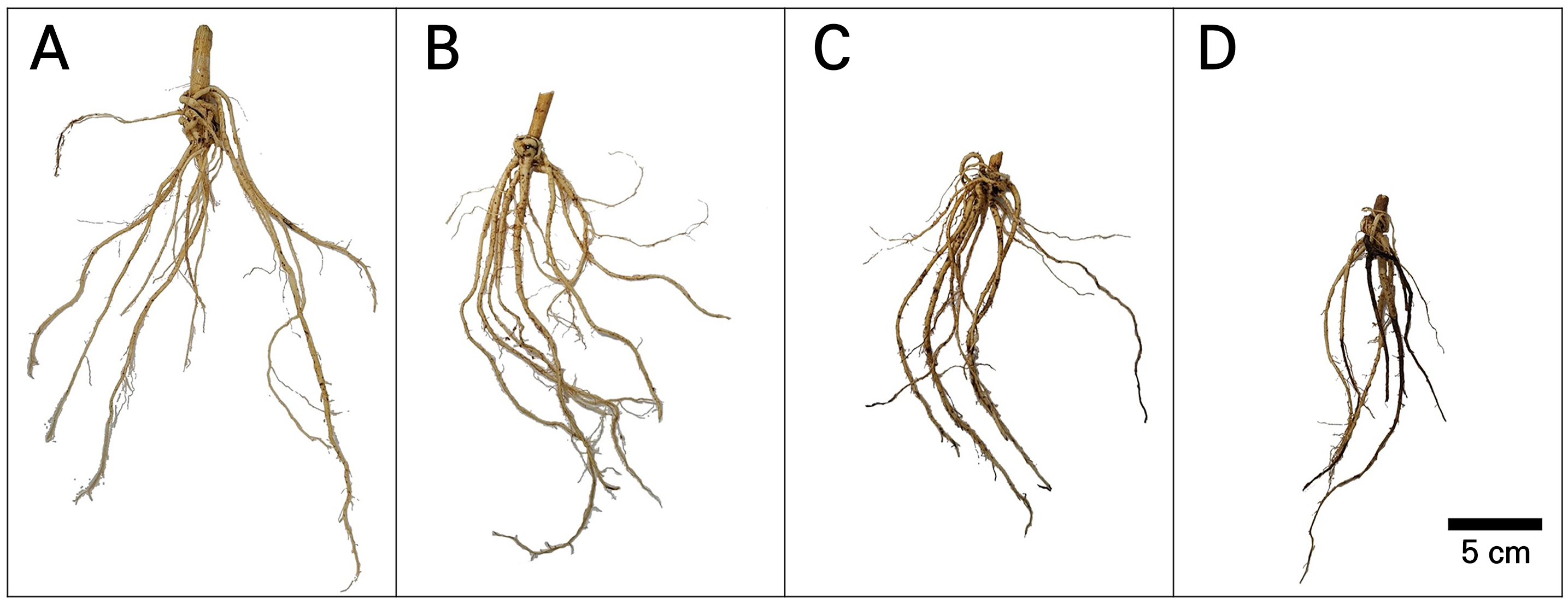
Fig. 7.
Representative picture images in the fresh root system after different temperature-stress experiments of Astragalus membranaceus grown in a temperature-gradient greenhouse. (A) T1, increase by about 0‒1.8°C from ambient temperatures; (B) T2, increase by about 2.3‒2.5°C from ambient temperatures; (C) T3, increase by about 3.3‒3.5°C from ambient temperatures; and (D) T4, increase by about 5.1‒5.3°C from ambient temperatures. The scale bar represents 5 cm.
Leaf surface microstructure
The leaf microstructure was observed by means of scanning electron microscopy (SEM) for each temperature range. The adaxial and abaxial surfaces of Hwang-gi leaves exhibited veins, trichomes, epicuticular wax, stomata, and roughness. The adaxial surface of T1 (control) showed clear morphological features with normal stomatal openings and a few trichomes around the veins (Fig. 8A and 8E). T2 showed morphological features similar to those of T1, with similar levels of stomatal opening and a few trichomes around the veins (Fig. 8B and 8F). T3 showed a significant increase in the number of trichomes, a decrease in the stomatal density, and a decrease in the stomatal size owing to the closure of many stomata compared to T1 and T2 (Fig. 8C and 8G). T4 showed a more pronounced decrease in stomatal size owing to the closure of many stomata. In addition, the leaf surface epidermal cells collapsed and condensed owing to a loss of turgor pressure, and the leaf surface wax was deformed (Fig. 8D and 8H). The increased number of trichomes, the stomatal closure, and the reduced stomatal density observed in the high-temperature groups, notably in T4, were evident strategies for minimizing the leaf surface moisture loss associated with the decreased relative humidity and soil moisture reduction due to the elevated temperatures.

Fig. 8.
Representative micromorphological variations on the adaxial (A, B, C, D) and abaxial (E, F, G, H) leaf surfaces of Astragalus membranaceus grown in a temperature-gradient greenhouse: (A, E) T1, increase by about 0‒1.8°C from ambient temperatures; (B, F) T2, increase by about 2.3‒2.5°C from ambient temperatures; (C, G) T3, increase by about 3.3‒3.5°C from ambient temperatures; (D, H) T4, increase by about 5.1‒5.3°C from ambient temperatures. Scale bars represent 500 μm. Note: Zoomed-in circles are high-magnification images of the surfaces and include the surface structures, trichomes, epicuticular wax deposits, leaf roughness, and stomatal size. Circle images are identical to the scale bars of the rectangle images and represent a length of 20 μm.
The epidermis is the outermost layer of all plant organs and serves as the first point of contact with the surrounding environment (Glover et al., 2016). Trichomes derived from the aerial epidermis are specialized structures that form protrusions on the leaf surface of plants and play a physiologically important role in plant-environment interactions (Glover et al., 2016; Galdon-Armero et al., 2018; Li et al., 2023). Additionally, the epidermis includes stomata, which are epidermal pores that regulate gas exchange and directly contribute to the regulation of the water status (Galdon-Armero et al., 2018). In particular, the control of the gas exchange between leaves and the external atmosphere is governed by stomatal conductance (Gs), and the stomata play an important role in photosynthesis, the transpiration rate, and the overall plant productivity (Lawson and Matthews, 2020). Therefore, the specialized structure of the epidermis is a promising target for improving plant drought resistance and water use efficiency (WUE) by limiting water loss (Franks et al., 2015; Bertolino et al., 2019; Waseem et al., 2021).
The role of trichomes in the resistance and adaptation to water stress has been reported in several plants. For example, Sletvold and Ågren (2012) showed that a polymorphic population of Arabidopsis lyrata that produces trichomes is associated with higher drought resistance than glabrous plants. Recent studies have shown that trichomes are important epidermal dew uptake structures that help to partially maintain leaf hydraulic conductivity, mitigate the negative effects of drought stress, and contribute to plant growth in dry environments.
Pharmacological characteristics
Temperature stress can induce many physiological, biochemical, and molecular changes in plant metabolism and can alter the production of secondary metabolites in plants (Zobayed et al., 2005). Hwang-gi roots boast a treasure trove of secondary metabolites, including triterpene-derived saponins (e.g., astragalosides) and isoflavonoids (e.g., calycosin, calycosin-7-O-glucoside, formononetin). Additionally, they harbor a diverse array of polysaccharides and amino acids, contributing to their unique composition and potential health benefits (Xiao et al., 2011; Zhang et al., 2014; Pan et al., 2015; Gai et al., 2017; Huang et al., 2018; Yang et al., 2019; Bao et al., 2022; Liang et al., 2023). Hwang-gi roots are currently receiving considerable attention owing to their various health benefits. Pharmacological analyses have shown promising improvements in the human immune system and sperm motility and in certain conditions, including chronic fatigue syndrome, kidney disease, diabetes, and hypertension (Zhang et al., 2011).
Following the temperature treatment, the marker compounds detected in Hwang-gi roots, as clearly identified in Fig. 9, were astragaloside I, astragaloside IV, formononetin, calycosin, calycosin-7-O-glucoside, and methylnissolin-3-O-β-d-glucopyranoside. The contents of formononetin, calycosin, and calycosin-7-O-glucoside were higher in T3 and T4 compared to those in T1 and T2 (Fig. 9A). In more detail, the elevated temperature (raised by 50% in the present study) significantly boosted the physiological activities of formononetin and calycosin-7-O-glucoside, raising them by 77% and 78%, respectively. Calycosin increased with the temperature, particularly exhibiting the most significant increase of 77% in T3. Conversely, methylnissolin-3-O-β-d-glucopyranoside showed the greatest decrease of 50% at T4 as the temperature was increased (Fig. 9A). Astragaloside I decreased with as the temperature increased, with the greatest decrease of 54% occurring in T3 (Fig. 9B). Interestingly, astragaloside IV levels did not exhibit a clear decreasing or increasing trend with an increase in the temperature (Fig. 9B). This suggests that this compound is not significantly affected by temperature stress. Formononetin, an isoflavonoid known to inhibit nitric oxide production and inflammatory enzymes (Lai et al., 2013; Ikbal et al., 2022; Dong et al., 2023), showed increased levels in T4-treated roots. Additionally, astragaloside IV, a crucial marker of pharmacological activity in Hwang-gi (Shaw et al., 2013; Wu et al., 2020; Liang et al., 2023), remained relatively stable across the temperatures tested here. Interestingly, formononetin, with its potential to antagonize astragaloside I, increased, whereas the latter decreased at higher temperatures. These findings lead us to believe that higher temperatures may negatively impact the biosynthesis or stability of astragaloside I in Hwang-gi roots. In this study, the marker compounds detected in Hwang-gi roots after the temperature treatment were astragaloside I, calycosin, methylnissolin-3-O-β-d-glucopyranoside, calycosin-7-O-glucoside, formononetin, and astragaloside IV, as clearly identified in Fig. 9. Among these, calycosin-7-O-glucoside and formononetin showed higher contents in T3 and T4 than in T1 and T2 (Fig. 9A), whereas astragaloside I showed significantly lower contents in the higher temperature ranges (Fig. 9B). Importantly, formononetin, an isoflavonoid, has been reported to gradually inhibit nitric oxide (NO) production and the expression of inducible NO synthase and cyclooxygenase (Lai et al., 2013; Ikbal et al., 2022; Dong et al., 2023). In this study, formononetin levels were significantly increased in Hwang-gi roots grown in the high-temperature treatment group T4.
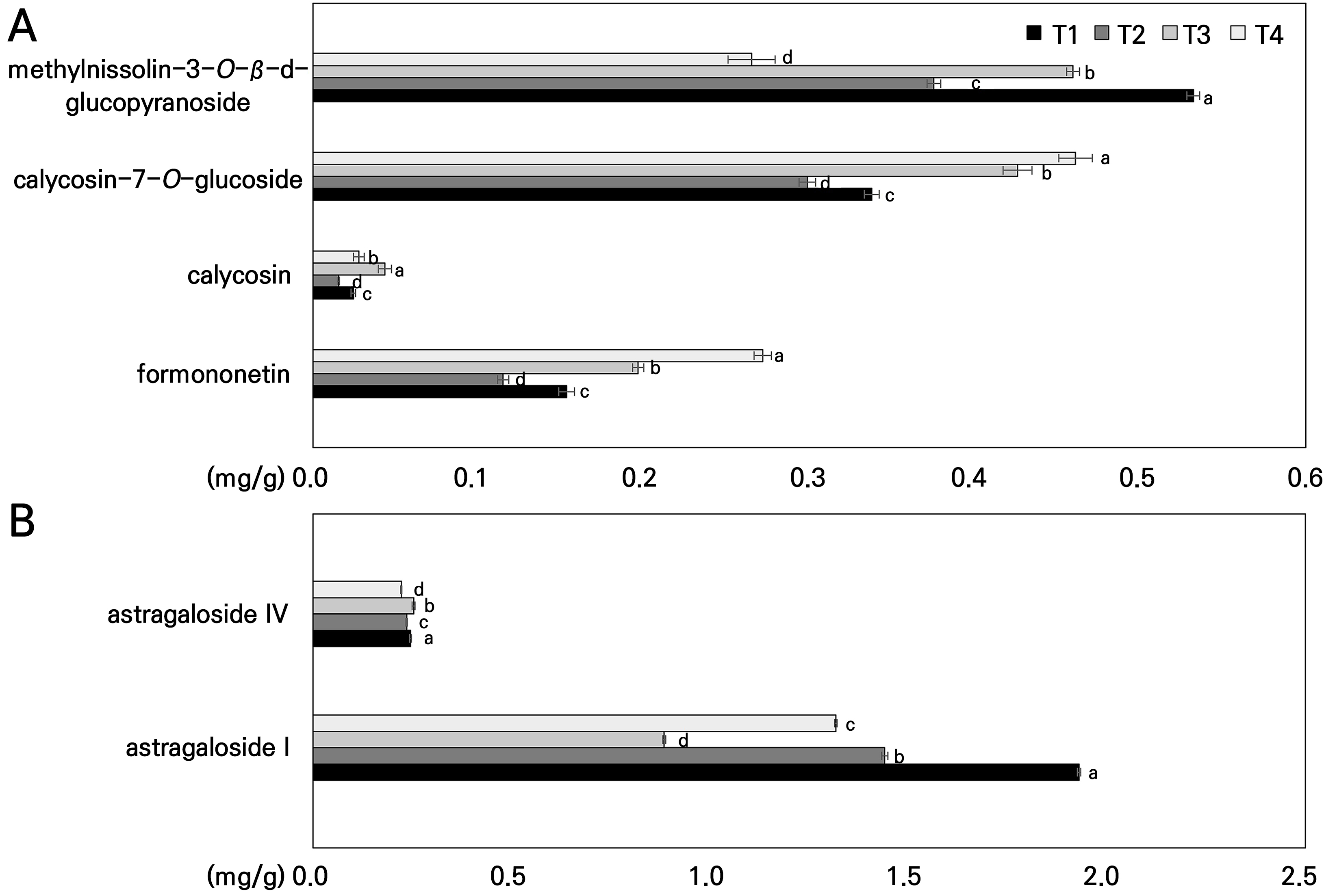
Fig. 9.
Influence of the temperature on the contents of (A) saponins (astragaloside I and astragaloside Ⅳ) and (B) isoflavonoids (calycosin-7-O-glucoside, methylnissolin-3-O-β-d-glucopyranoside, formononetin, and calycosin) accumulated in the hairy roots of Astragalus membranaceus grown in a temperature-gradient greenhouse based on climate change scenarios. Data represent the means ± SD of 3 replicate roots. Different letters on the bars denote significant differences (p < 0.05). T1, T2, T3, and T4 represent each temperature section in the temperature-gradient greenhouse depicted in Figure 1.
Notably, astragaloside IV, a cycloartane-type triterpene glycoside, is recognized as a fundamental marker compound used to access the pharmacological attributes of Hwang-gi extract (Shaw et al., 2013; Wu et al., 2020; Liang et al., 2023). The roots of Hwang-gi are currently attracting considerable attention owing to their various health benefits. Pharmacological analyses have shown promising improvements in the human immune system and in sperm motility, chronic fatigue syndrome, kidney disease, diabetes, and hypertension (Zhang et al., 2011).
In the present study, the biomass of Hwang-gi grown at high temperatures was significantly decreased by more than 50% (Fig. 4). Interestingly, while the root diameter decreased with the rising temperature (Fig. 7), certain indicator components in Hwang-gi roots, in this case calycosin-7-O-glucoside and formononetin, exhibited an upward trend (Fig. 9). This is consistent with the correlation between the growth of Hwang-gi roots and the pharmacological components (Yu et al., 2007; Chen et al., 2021; Chien et al., 2022). Astragaloside IV and the flavonoid contents in Hwang-gi roots generally decrease as the root thickness increases and are strongly correlated with the root diameter (Yu et al., 2007; Chien et al., 2022).
The pharmacological quality of Hwang-gi is closely related to ecological factors such as climate, soil, and cultivation years in the production area (Li et al., 2017; Yang et al., 2019; Chen et al., 2021). Li et al. (2017) demonstrated a close correlation between climatic factors and chemical constituents, suggesting that climatic factors are closely associated with the quantities of chemical components. Astragaloside showed a weak positive correlation with the wind speed, solar radiation, and evaporation, and a weak negative correlation with precipitation, the relative humidity, and the minimum temperature. Formononetin and calycosin, on the other hand, showed a strong negative correlation with the wind speed, sunshine hours, and evaporation and a strong positive correlation with precipitation, the relative humidity, and the minimum temperature. Moreover, as indicated by Lin et al. (2022), cultivated Hwang-gi faces water scarcity threats at all growth stages and exhibits significantly lower levels of pharmacological constituents than wild-harvested Hwang-gi throughout its growth phase. Yang et al. (2020) identified temperature and precipitation as the key ecological drivers of the growth and astragaloside IV content of Hwang-gi (Astragalus membranaceus var. mongholicus) in Inner Mongolia. Conversely, the factors of the sunshine duration and soil pH were found to govern calycosin-7-O-glucoside levels primarily. These findings highlight the crucial role of environmental factors in optimizing Hwang-gi cultivation. Adjustments in temperature and precipitation regimes offer promising avenues for boosting astragaloside IV, while enhanced sunshine and soil pH manipulation can elevate calycosin-7-O-glucoside production. Furthermore, Feng et al. (2021) found that drought stress stimulated the accumulation of methyl jasmonate (MeJA), which is involved in drought-stress-induced calycosin-7-O-glucoside production in Hwang-gi roots; an exogenous application of MeJA effectively enhanced the calycosin-7-O-glucoside content of Hwang-gi roots. Hwang-gi roots frequently encounter drought, strong light, ultraviolet radiation, and nutrient deficiency stress. This suggests that through natural selection, they have evolved towards the biosynthesis of secondary metabolites (Guo et al., 2020).
Conclusion
This study provides valuable insights into the multifaceted responses of Astragalus membranaceus (Hwang-gi) under conditions of elevated ambient temperatures associated with limited soil moisture and heat stress. This study sheds light on the growth, physiological characteristics, morphological changes, and pharmacological responses to temperature stress of this plant, finding that increasing atmospheric temperatures accelerate surface drying and reduce soil moisture, possibly leading to reduced water absorption and poorer water use efficiency and photosynthesis owing to stomatal closure, highlighting the vulnerability of Hwang-gi during medicinal plant cultivation. This study suggests that Hwang-gi exposed to environments with elevated temperatures may have reduced growth and survival rates of the aerial parts and decreased root yields. Additionally, high temperatures were found to induce changes in the leaf surface microstructure, specifically increased numbers of trichomes and decreased stomatal density levels, indicating a plant adaptation strategy to mitigate water loss. The temperature significantly altered the pharmacological profile of Hwang-gi roots by affecting the content and potential activity of key marker compounds. Meanwhile, we speculate that the synergistic or antagonistic effects of formononetin may have the same promoting or inhibiting effect on astragaloside I activity at elevated temperatures. This necessitates further investigations to understand the full impact of temperature stress on the medicinal properties of this plant. Understanding these responses is important for Hwang-gi and for other medicinal plants so that growers can adapt these plants to climate change while maintaining high-quality medicines. Consequently, the findings here not only contribute to a better understanding of the adaptive strategies of plants in response to climate change and to the creation of strategies for future agriculture and ecosystems but also emphasize the importance of considering environmental factors for the cultivation and enhancement of medicinal plant quality.