Introduction
Materials and Methods
Experimental plot layout
Treatment applications
Soil and leaf mineral nutrient analyses
Bush performance
Data analysis
Results and Discussion
Soil and leaf mineral nutrients
Bush performance
Conclusions
Introduction
Globally, plants of the genus Aronia of the Rosaceae family, commonly known as chokeberries, are divided into three types as per their color: the purple chokeberry (Aronia prunifolia), black chokeberry (Aronia melanocarpa (Michx.) Elliot), and red chokeberry (Aronia arbutifolia (Michx.) Elliot) (Brand, 2010; Strik et al., 2003). Chokeberries grow naturally in North America and are primarily cultivated in Poland, which accounts for approximately 90% of the world’s production (Strik et al., 2003; Brand, 2010). Black chokeberries are referred to as a functional food due to the highest compounds of phenolics, flavonoids, and anthocyanins, as well as antioxidant activities among the fruit species (Kokotkiewicz et al., 2010; Choi, 2020a; Zhang et al., 2021; Đorđević et al., 2022; An et al., 2023; Paunović et al., 2023; Strik et al., 2003), leading to production approximately 100 times higher in 2018 than in 2013 in South Korea (Wonye, 2023). However, the main black chokeberry cultivar, Nero, is consumed primarily in the form of a powder due to the strong tart taste of the fresh berry and have largely been imported at a low price from Eastern Europe since 2016 (Won et al., 2017; Paunović et al., 2023; Wonye, 2023). Furthermore, shortages in farm labor and labor expenses have rapidly increased since the COVID-19 pandemic in 2020, resulting in high production costs and decreased farm revenue and cultivation areas.
Organic fruits and vegetables have been more profitable than non-organic products owing to increasing concerns about food safety and the environment for farmers, consumers, and the government since late the 1990s (Hwang, 2009; Reganold and Wachter, 2016; Choi, 2020a, 2020b). The domestic production of organic black chokeberry has been a priority to compensate for the decrease in net income through high premium prices for growers as well as the competition with imported conventional berry powder products (Choi, 2020a; Đorđević et al., 2022). Organic farming systems do not allow for the application of synthetic chemical fertilizers and pesticides, allowing instead for the use of naturally derived sources legally required by national administrations (Luttikholt, 2007; Choi, 2020a, 2020b; Đorđević et al., 2022). Oil cake, a by-product of the residue of crushed seeds from soybean, rapeseed, oil palm, flax seeds, and mixed seeds, which has been excessively applied as a basal fertilizer to supply nutrients to organic plantations in South Korea over the last few decades (An et al., 2012; An et al., 2019; Choi, 2020a, 2020b; Choi and Jung, 2020; Jung and Choi, 2020). Alternative nutrient sources should be developed to reduce the over-reliance on the application of oil cake imported from North America and to induce a proper nutrient balance in the soil.
A large number of starfish (Asterias amurensis) now exist in the ocean, washing ashore along the coastline due to global warming since the 2000s while others have been abandoned by some fisherman without authority, causing marine and coastal contamination as well as biodiversity loss (Ishii et al., 2007; An et al., 2012; An et al., 2019; Choi, 2020b; Bae et al., 2023). Waste starfish is recycled for supplementation with various field crops to improve soil nutrition and soil ecosystems as an additional liquid fertilizer with high levles of biological active compounds (Ishii et al., 2007; An et al., 2012; An et al., 2019; Kuk et al., 2019; Choi, 2020a, 2020b; Jung and Choi, 2020; Bae et al., 2023). However, there is limited information available on chokeberry production combined with soil environment fertilized with starfish waste during the growing season. This study was initiated to determine the optimum nutrient source for oil cake and starfish to maximize the performance and fruit productivity of organic black chokeberry over two years.
Materials and Methods
Experimental plot layout
The study was conducted at the University Experimental Farm Station located in Gyeongsan-si, South Korea (35°N/128°49’E) from 2020 (year 1) to 2021 (year 2). The experimental plots were ensured to be environmentally friendly and had been cultivated with various vegetables and ornamental crops using low chemical inputs for five years. The soil texture between 0 and 30 cm depths in the root zone was mostly loamy sand, comprising 57.2% sand, 34.0% silt, and 8.9% clay.
One-year-old ‘Nero’ black chokeberry bushes were planted at a density of 2.0 m between the bushes and 4.0 m between rows in 2018. All of the black chokeberries were grown naturally without thinning of the shoots as small-sized bushes with a height of 50–100 cm during the experimental period. A hand-held hose was used to irrigate the rooting zone uniformly in the absence of rain for more than five consecutive days during the growing season. Black polypropylene woven mulch was used to suppress weed growth and conserve the soil moisture around the base of the bush. The average temperature and accumulated precipitation from April to August were correspondingly 21.7 °C and 909.0 mm in year 1 and 21.7°C and 598.6 mm in year 2, with mean values of 22.1°C and 746.9 mm between 1991 and 2020 (KMA, 2021). Insects and diseases were managed with organic pesticide applications during the growing season.
Treatment applications
The experimental unit (data unit) was comprised of a single chokeberry, and three black chokeberry plants were randomly arranged in each treatment. Four different treatments were assigned with 0% pelletized oil cake with starfish (0OC+SF), 33% oil cake with starfish (33OC+SF), 66% oil cake with starfish (66OC+SF), and 100% oil cake (100OC). Here, 0OC, 33OC, 66OC, and 100OC indicate the application of oil cakes at 0, 33, 66, or 100% of the recommended annual rate, respectively, equivalent to approximately 14 g of total nitrogen (T-N) tree-1 for the T-N requirement for blueberry production according to the protocols of the Rural Development Administration (2015).
The OC materials comprised 5.6% T-N, 0.6% P, 0.1% K, 0.39% Ca, 0.35% Mg, and 70% organic matter (Chamjoa, Farmhannong Co., Seoul, Korea). The fermented starfish fertilizer comprised primarily starfish extract at 40.3%, seaweed at 10.1%, and molasses at 3.4%, with additional mixing with effective microorganisms at a rate of 0.2%, humus at 0.8%, and water at 45.2% over three years, containing 0.0044% T-N, 0.002% P, 0.122% K, 0.311% Ca, and 0.142% Mg (Choi, 2020a, 2020b). The OC fertilizers were scattered evenly around the bush before irrigation in April of each year. The 100-fold diluted SF was fertigated on the soil surface using a plastic cup from April to May, and weekly sprayed took place with a foliar application of approximately 1,000 mL of water eight times between June and July.
Soil and leaf mineral nutrient analyses
Soil samples were collected from a depth of 0–20 cm in the rooting zone with a soil auger at a distance of 25 cm from the main cane of each treatment bush to analyze the mineral nutrient concentrations in August, 90 days after full bloom (DAFB) of each year, following the protocols of the Rural Development Administration (2010). The soil samples were dried and sieved into fractions finer than 2.0 mm. The soil pH and electrical conductivity (EC) were measured at a 1:5 (w/v) soil-to-water ratio using a portable HI 2315 conductivity pH/EC meter (Hanna Co., Seoul, Korea). The soil organic matter (OM) contents were determined by oxidation using the Tyurin method. The concentrations of T-N and available P2O5 in the soil samples were analyzed using an elemental analyzer (Vario MAX CNS/CN, Elementar Trading Co., Ltd., Langenselbold, Germany) and differential spectrophotometry (UV-2450, Shimadzu, Tokyo, Japan), respectively. The soil-exchangeable concentrations of K, Ca, and Mg were analyzed using atomic absorption spectrometry (ICP-OES, SPECTRO Analytical Instruments DMC GmbH Co., Hamburg, Germany).
A total of 40 mature leaves from each bush were randomly collected at 90 DAFB of each year and were measured for their average leaf area, leaf perimeter, and specific leaf area (SLA) using a portable leaf area meter (CI-202, CID Bio-Science Co., Camas, USA). Leaf samples were dried in a dry oven at 65°C for three days and weighed using an electronic scale (PFB2000-2, Kern-Sohn GmbH, Balingen, Germany) to measure the leaf dry weight (DW). The leaf samples were then ground in a blender with four mill blades (WDL-1, Wonder Blender Co., Tokyo, Japan) to analyze their macro-nutrient concentrations (RDA, 2010). The Kjeldahl digestion method was used to determine the foliar concentration of T-N, the ammonium vanadate method served to determine the concentration of P, and atomic absorption spectrometry was used to determine K, Ca, and Mg.
Bush performance
Portable SPAD measurements (SPAD 502 chlorophyll meter, Minolta, Tokyo, Japan) were taken monthly from April to September of each year to read the chlorophyll concentrations in healthy green leaves on shoots.
The bush height, number of canes, the sum of the cane diameters, the canopy volume, the number of new shoots, the shoot length, and the number of leaves were recorded in mid-October (180 DAFB) in both years when there was little increase in the shoot growth. The number of canes was counted on each bush, and each cane diameter was measured 5.0 cm above the ground surface using a digital Vernier caliper to calculate the sum of the bush diameters on each bush. The average canopy volume was estimated by multiplying the canopy length and width by the height of each bush.
The interior light intensity was measured using a portable PPFD meter (J&C Technology Co., Ltd., Gimcheon-Si, Korea) from 12:00 to 14:00 pm at 60 DAFB in year 2 to determine the extent of light intensity at five different points 50 cm from the cane and above each bush.
Fruits were harvested twice at maturity and counted, and the fresh weight (FW) was used to calculate the fruit yield during years 1 and 2. In total ten fruits were randomly collected from each treated bush to analyze the fruit quality during the second fruit harvest in year 2. The fruit diameter and length were assessed using a digital caliper with accuracy of 0.01 mm (Mitutoyo Corp., Takatsu-ku, Japan). Fruit juice was extracted from the middle point of each side of the fruit to observe the soluble solid contents (SSC) using a handheld digital refractometer (GMK-706R, G-WON Hitech Co., Ltd., Seoul, Korea). The fruit surface color was expressed through the CIELAB color space, where L* indicates lightness, a* indicates the coordinates green to red, and b* indicates the coordinates blue to yellow, using a simple type of spectral colorimeter (FR-5105, X-Rite, Inc., Grand Rapids, USA).
The total anthocyanin content of the flesh samples was estimated according to the modified pH-differential method (Lee et al., 2005) through a colorimetrical analysis in a wavelength range of 510 nm using a UV-Vis-NIR spectrophotometer (Shimadzu/UV-1800, Shimadzu Scientific Instruments, Inc., Kyoto, Japan).
Whole black chokeberry bushes, roots, stems, and canes were completely harvested using a shovel in year 2 and were dried in a dry oven at 65°C for ten days to measure the DW of each organ and the corresponding dry matter partitioning. The fruit yield efficiency was determined as a percentage of the DW of the fruit divided by the DW of the whole bush.
Data analysis
Treatment means were compared between the groups by means of an analysis of variance. All statistical analyses were performed using SAS software (version 9.4, SAS Institute Inc., Cary, USA). Duncan’s multiple-range test was used for the mean separation of variables for each treatment at a significance level of 0.05.
Results and Discussion
Soil and leaf mineral nutrients
Total amounts of T-N in an average of two experimental years were annually applied to 17,024 mg for a 100OC treatment bush, 11,326 mg for a 66OC+SF bush, 5,670 mg for a 33OC+SF bush, and 70 mg for a 0OC+SF bush (Table 1). The total amounts of P supplied to the bush in each case were similar to the 100OC (1,324 mg) and 66OC+SF (1,238 mg) treatments. The 100OC treatment supplied lower amounts of K, Ca, and Mg compared to the 0OC, 33OC, or 66OC cases with SF containing concentrated physiologically active substances (Choi, 2020b; van der Heide et al., 2021; Bae et al., 2023).
Table 1.
Nutrient contents in fertilizers of oil cake (OC) and starfish (SF) used in an experiment plot during the growing season in Gyeongsan-si, South Korea
The soil pH in the 0OC+SF treatment plots increased to 7.3 in year 1 and to 7.1 in year 2 while the 100OC treatment reduced the pH levels, though remaining at desired levels to sustain annual bush growth for two years (RDA, 2015; Table 2). The oil cake fertilized in the 100OC plots would have mineralized into inorganic acids, HNO3 or H2SO4, leading to the bacterial nitrification of NH4 to NO3 (Bryla and Machado, 2011; Kim et al., 2015; Choi, 2020a; Choi and Jung, 2020) and causing soil acidification, which was observed in all plots with repeated fertilization in year 2. Soil EC was similar in all treatment plots three months after fertilization in either year. The concentrations of soil OM, T-N, and P2O5 were not significantly different between the treatment plots in either year. Soil OM demonstrated lower levels in all the treatment plots in both years compared to the recommended range of 25 to 35 g kg-1 to maintain optimum bush growth (RDA, 2015), but it increased only slightly from year 1 to 2. The 0OC or 33OC+SF treatment decreased the concentrations of soil K2O for two years but increased the CaO concentrations as a result of the competition among the cations to remain on the soil surface of the particles and colloids (Rietra et al., 2017; Choi, 2020a; Choi and Jung, 2020).
Table 2.
Soil mineral nutrition in a ‘Nero’ black chokeberry field with oil cake (OC) and starfish (SF) applied in Gyeongsan-si, South Korea
Table 3.
Leaf macronutrient concentration in a ‘Nero’ black chokeberry bush with oil cake (OC) and starfish (SF) applied in Gyeongsan-si, South Korea
The concentrations of T-N in the leaves decreased in the 0OC-treated bushes or the 33OC+ SF-treated bushes in years 1 and 2, and were lower than the corresponding optimum levels of 1.8% to 2.3% in year 2 when supplying low amounts of T-N from the fertilizers used (RDA, 2015; Table 3). The 66OC+SF or 100OC treatments did not increase the leaf concentrations of P, K, Ca, or Mg in either year, particularly for both P and Ca in year 2, possibly due to the effects of nutrient dilution on the increased DW in the treated bushes (Davis, 2009), which was responsible for the decrease in the foliar concentrations of T-N and P from year 1 to year 2. Leaf Ca concentrations of all bushes were much greater than the optimum level in both years (RDA, 2015), influenced by calcareous soils in the experimental plots (Rietra et al., 2017), which can be adjusted upwards for Ca requirements to maintain annual bush growth.
Bush performance
Leaf SPAD readings were greater for the 66OC+SF and 100OC treated bushes in August in year 1 and June and September in year 2 (Fig. 1A and 1B). Seasonal SPAD variations increased from April to August in both years. An increase in the amount of fertilizers containing mineral nutrients, particularly T-N, would have induced the formation of chlorophyll molecules and photosynthetic responses in the bushes during this season (Williamson and Miller, 2009; Jeppsson, 2000; Bryla and Machado, 2011; Kwack et al., 2017); An increase in SPAD was, however, not observed in September due to the nutrient remobilization of the canes from the leaves. High SPAD values are favorable in the late season for sustaining photosynthetic effects, reserving more carbohydrates, protecting against spring frost damage, and increasing the regrowth of chokeberries in the following season (Huett, 1996; Leitzke et al., 2015; Choi, 2020a).
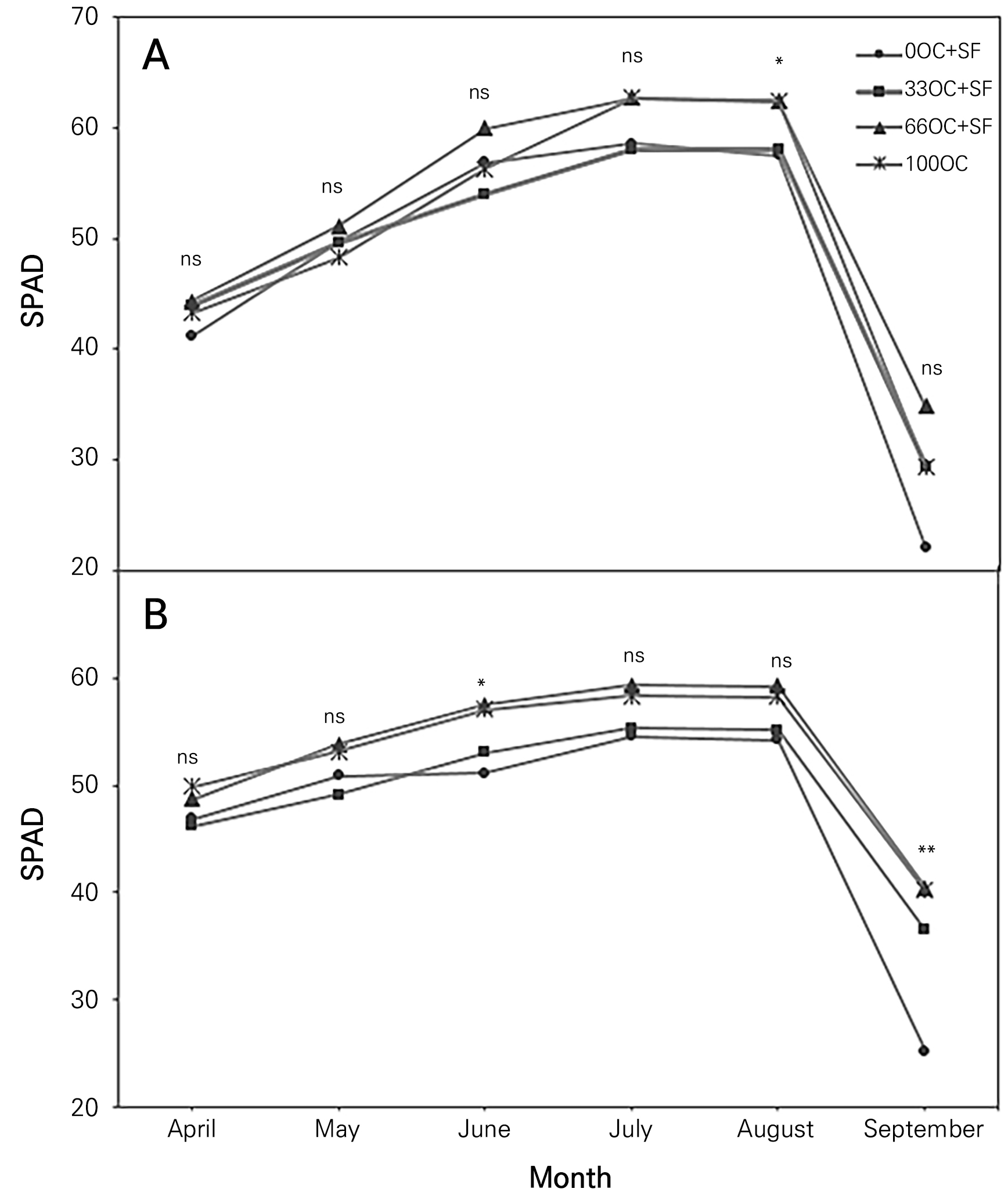
Fig. 1.
Seasonal SPAD values of a ‘Nero’ black chokeberry bushwith oil cake (OC) and starfish (SF) applied in Gyeongsan-si, South Korea in year 1 (Panel A) and year 2 (Panel B). OC: oil cake, SF: starfish. 0-, 33-, 66-, and 100-OC correspondingly indicate oil cake applications at 0, 33, 66, or 100% of the recommended annual rate, equivalent to approx. 14 g N tree-1. * and ** adjacent to each datum point indicate significant differences according to Duncan’s multiple-range test at p < 0.05 and < 0.01, respectively; ns, not significantly different.
The bush height was increased by the 100OC treatment in year 1 but not significantly different among the treatment bushes in year 2 (Table 4). The cane width and length were significantly expanded by the 100OC treatment in both years, producing a large canopy volume, being representative of the canopy occupation of blueberry (Williamson and Miller, 2009; Kwack et al., 2017; Đorđević et al., 2022). The 0OC+SF treatment significantly reduced the number of canes and the sum of the cane diameters, an indicator of the aboveground vegetative biomass of highbush blueberry, followed by 33OC+SF, which would have decreased the number of shoots and leaves and the length of the shoots in these bushes (Pritts and Hancock, 1985). The 66OC+SF treatment did not significantly increase vegetative growth, most likely due to the production of high fruit yields (Fig. 2). Substantial vegetative growth of the 100OC bushes, followed by the 66OC+SF-treated bushes, would cause these plants to receive relatively lower light intensity of 343 lx inside the canopy in year 2. Regarding the light intensity, this was similarly observed in the canopy of persimmon fruit trees treated with different application rates of oil cake (Kim et al., 2015).
Table 4.
Growth of a ‘Nero’ black chokeberry bush with oil cake (OC) and starfish (SF) applied in Gyeongsan-si, South Korea
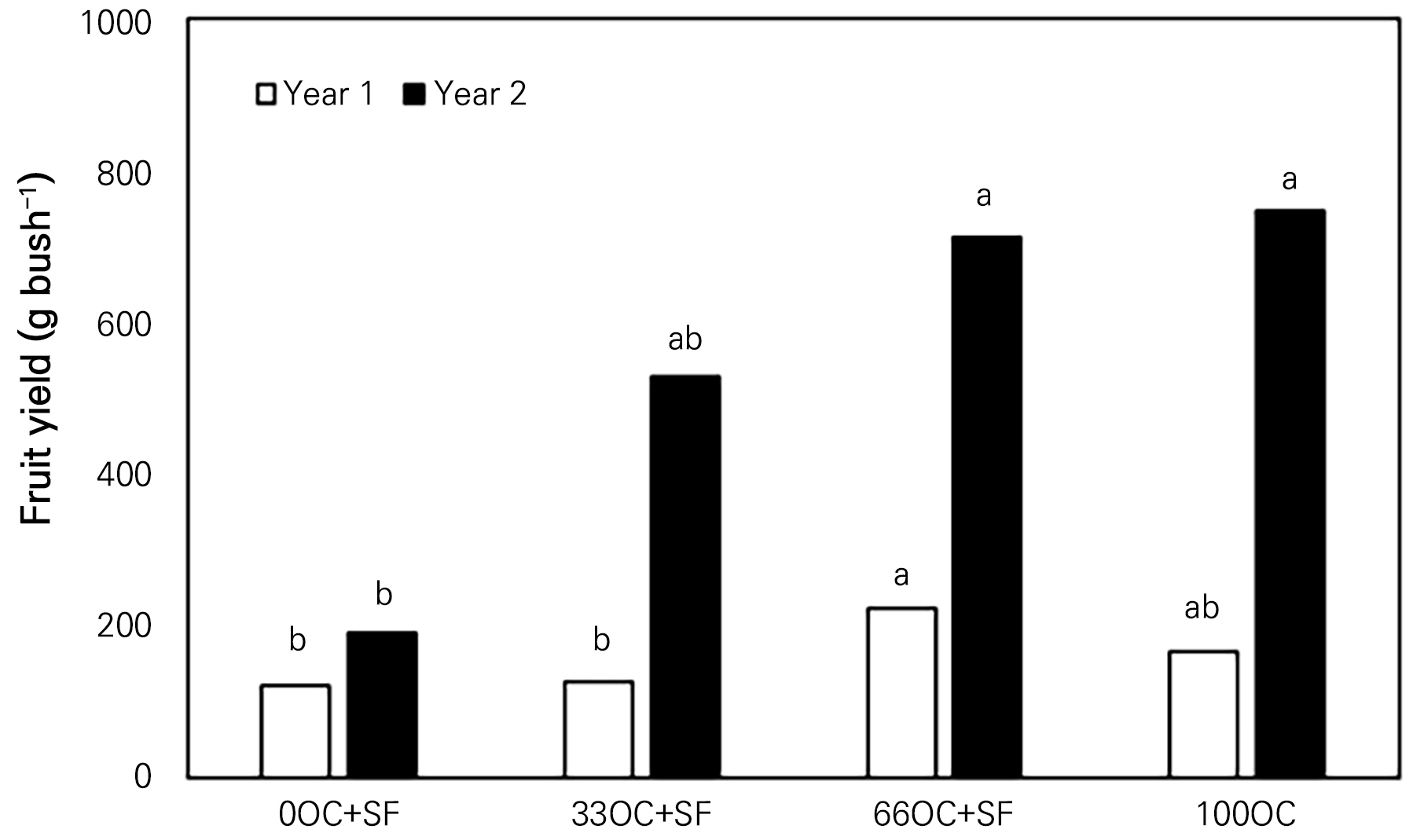
Fig. 2.
Fruit yield of a ‘Nero’ black chokeberry bush with oil cake (OC) and starfish (SF) applied in Gyeongsan-si, South Korea in year 1 (Panel A) and year 2 (Panel B). OC: oil cake, SF: starfish. 0-, 33-, 66-, and 100-OC correspondingly indicate oil cake applications at 0, 33, 66, or 100% of the recommended annual rate, equivalent to approx. 14 g N tree-1. Different lowercase letters indicate significant differences at each datum point according to Duncan’s multiple-range test at p < 0.05.
The total leaf areas, rated from highest to lowest, were observed in the 100OC-treated bushes, the 66OC+SF bushes, and the 33OC+SF bushes in year 2; these were affected by the amounts of vegetative growth (Table 5). There were, however, no significant differences observed in the average leaf DW, leaf area, leaf perimeter, or SLA between the treated bushes, presumably due to the dilution effects of the larger bushes.
Table 5.
Leaf characteristics of a ‘Nero’ black chokeberry bush with oil cake (OC) and starfish (SF) applied in Gyeongsan-si, South Korea in year 2
Yields of organic black chokeberry or blueberry are proportionally increased with an increase in the canopy volume and height due to the increased rates of fertilization (Williamson and Miller, 2009; Rietra et al., 2017; Đorđević et al., 2022). However, the 66OC+SF treatment here showed increased fruit yields in both years, with the second highest level observed for the 100OC treated bushes (Fig. 2A and 2B), as the SF fertilizer contained a high protein fraction as well as carbohydrates and minerals, aiding in plant growth and development (Choi, 2020b; van der Heide et al., 2021).
The DWs of the root, stem, cane, and fruit were the greatest for the 100OC treatment bushes in year 2, followed by the 33OC+SF, 66OC+SF, and 0OC+SF bushes (Fig. 3). The fruit yield efficiency, however, improved up to 13.6% for the 66OC+SF bushes, up to 12.4% for 100OC, up to 8.5% for 33OC+SF bushes, and up to 6.0% for 0OC+SF bushes (data not presented). This would have resulted from the promotion of top growth of the 66OC+SF bushes, particularly for the reproductive stem parts of the whole tree, as was similarly observed in ‘Viking’ chokeberries and cranberries after a proper amount of fertilizer was applied (Jeppsson, 2000).
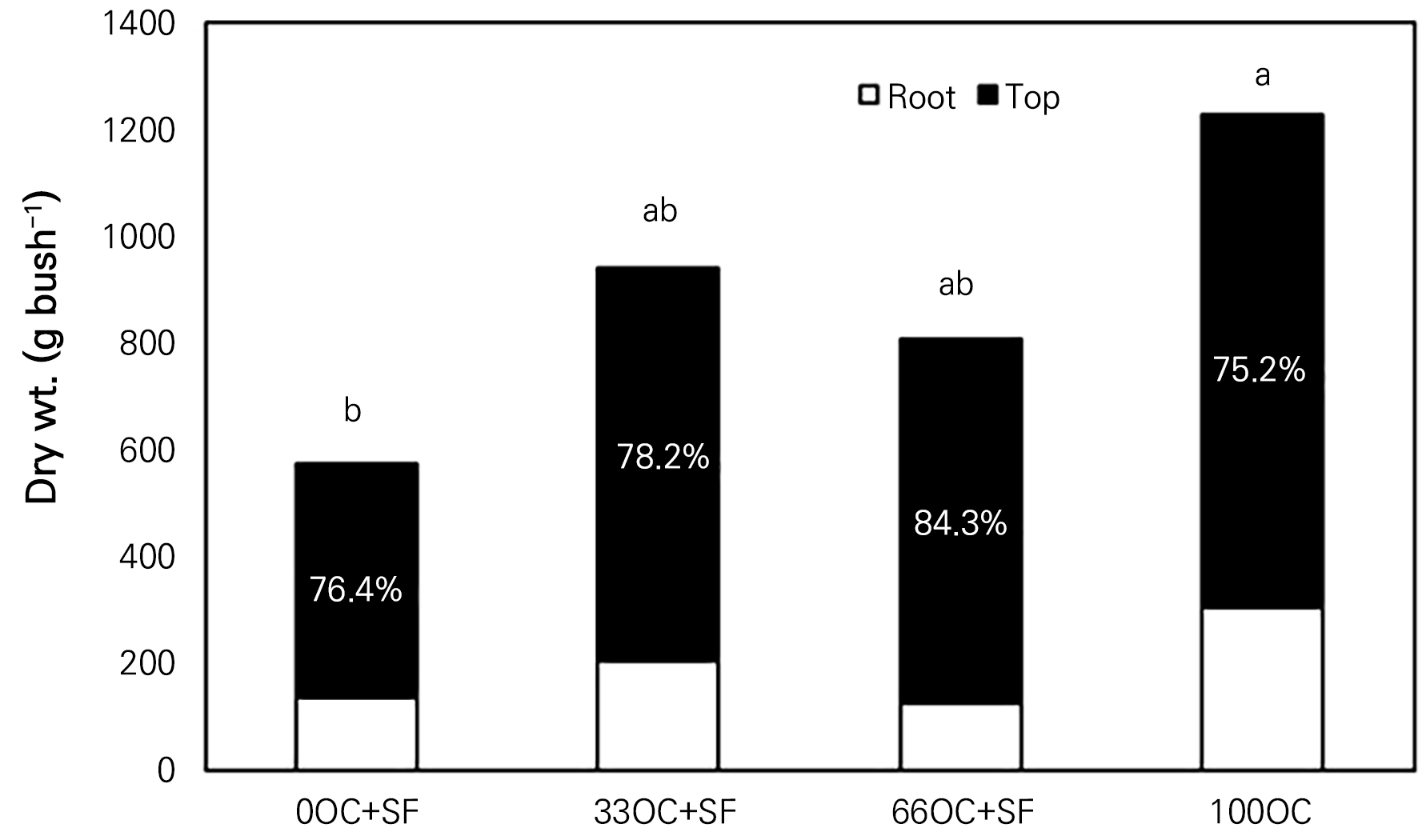
Fig. 3.
Dry matter partitioning of a ‘Nero’ black chokeberry bush with oil cake (OC) and starfish (SF) applied in Gyeongsan-si, South Korea in year 2. OC: oil cake, SF: starfish. 0-, 33-, 66-, and 100-OC correspondingly indicate oil cake applications at 0, 33, 66, or 100% of the recommended annual rate, equivalent to approx. 14 g N tree-1. Different lowercase letters indicate significant differences at each data point according to Duncan’s multiple-range test at p < 0.05.
The 0OC+SF treatment resulted in reduced fruit lengths and fruit diameters but led to a high L:D ratio in year 2 (Table 6), most likely due to the lack of cell enlargement given the supply of low amounts of mineral nutrients during the later growth stages of the fruit (Ozga and Reinecke, 2003). The fruit FW and SSC were observed to increase in the 33OC+SF, 66OC+SF, and 100OC treatments, with no significant difference observed in the fruit acidity. The amount for 33OC+SF would be sufficient to enhance the fruit size and taste, with much higher supplementation of K, Ca, and Mg elements in SF compared to the 100OC treatment, in good agreement with previous results on fruit productivity of tomato plants treated with starfish fertilizers (Choi, 2020b; Bae et al., 2023). This may have stimulated genetic materials in the form of DNA and RNA as well as cell membrane components, carbohydrate synthesis, and transports to increase fruit sizes and sugar contents (Marschner, 2012; Choi, 2020b). The 33OC+SF, 66OC+SF, and 100OC treatments significantly decreased the fruit surface color of lightness (L*) and yellow (b*), with an increase in the color red (a*) observed for the 66OC+SF and 100OC treated bushes, associated with the advanced ripening of the fruit caused by the increased fertilizer level. The 33OC+SF treatment enhanced the total anthocyanin contents of the fruits, receiving a high amount of light intensity (An et al., 2023), with this result similar to that of the 100OC treatment fruits. The low T-N fertilizer rates, in this case for 33OC+SF, were primarily due to the accumulation of anthocyanins rather than to the biosynthesis of other substances in the fruits (Jezek et al., 2018; Jeppsson, 2000; Đorđević et al., 2022). Synthesis of anthocyanin contents in fruits was reduced by the 0OC+SF treated bushes, with this being asscoiated with warm and dried soil conditions reported from Won et al. (2017) and Paunović et al. (2023).
Table 6.
Fruit characteristics of a ‘Nero’ black chokeberry bush with oil cake (OC) and starfish (SF) applied in Gyeongsan-si, South Korea in year 2
Conclusions
The 66OC+SF treatment is an alternative nutrient source to be considered in place of 100OC in organic black chokeberry given its moderately high levels of leaf T-N and relatively high SPAD values, the medium-sized production of bushes, and the high fruit yields. The 33OC+SF treatment improved the overall fruit quality to some extent by promoting the nutrient availability of starfish, which primarily consisted of polysaccharide seaweeds and plant growth stimulants (An et al., 2012; Hernández-Herrera et al., 2014). There is, however, a lack of information on the sustainability of soil agroecosystems and berry antioxidants from oil cake supplements combined with starfish or other organic nutrient sources; such information is necessary for the long-term assessment of soil properties and overall tree production in repeated field experiments under year-to-year variations.